Final report for GNC20-310
Project Information
While agricultural soils account for a large portion of nitrogen pollution in the US, sustainable soil management practices present unmatched potential to slow global warming and counteract environmental externalities. Planting cover-crops is part of a set of recommended practices in conservation agriculture, a management approach that encourages farmers to decrease N losses by keeping living plants and plant cover in the soil during the off-season. Despite their promise in reducing sources of N loss through denitrification and leaching, the functional impact of cover-crops on microbially-driven soil N-cycling remains a mystery. At a time when cover-crops are gaining increased interest across the country, including in the Upper Midwest, leveraging below-ground interactions with microbes to tighten nutrient cycles presents the next big challenge for climate-friendly farming.
This project contributes to an existing SARE project aimed at engaging minority farmers in summer cover crop systems. Under the current project, optimal management of a variety of summer cover crop species is being investigated to maximize ecosystem services such as providing habitat for pollinators and plant-available mineral N. The objective of this proposal is to understand how summer cover-crop management impacts key soil microbial groups.
Cover crop management decisions such as species selection and termination date can influence microbial groups responsible for transforming soil N in ways that are not well-understood. Agriculturally-relevant sources of N loss that contribute to water pollution and greenhouse gas emissions are controlled by the key microbial processes of nitrification and denitrification. The objectives of this project are to 1) identify summer cover crop species and species mixtures that minimize soil N loss via impacts on key microbial communities, and 2) contribute to resources for underserved farmers to assess summer cover crop management trade-offs for ecosystem services. Farmer collaborators will assist in evaluating seven cover crop species mixture treatments, each at two different planting dates. Soil samples will be collected from on-farm trials at key timepoints to determine cover crop impacts on microbial populations. Information on how cover crop management interacts with microbial communities will be paired with qualitative farmer evaluation of the cover crop systems under study. This project will ultimately engage small-scale vegetable producers and minority farmers in making informed decisions on the unseen, below-ground impacts of planting cover-crops.
Objective 1. Identify summer cover crop species and species mixtures that minimize soil N loss through impacts on specific microbial communities. Learning outcome: 5 educators/researchers will learn about the impact of at least three summer cover-crop species on soil microbial groups that play a central role in soil N cycling. Action outcome: Educators will develop and distribute recommendations to 200 farmers for using summer-cover crops to functionally impact soil microbial communities.
Objective 2. Contribute to resources for immigrant and minority farmers to assess management trade-offs with summer cover crop ecosystem services. Learning outcome: 50 growers will learn to weigh impacts on soil microbial populations in their decision to adopt summer cover crop mixtures. Action outcome: Growers contribute to the preservation of plant-available soil N and reduce losses from leaching and denitrification by cultivating beneficial microbial communities through cover crop selection.
Cooperators
- (Researcher)
- (Researcher)
- (Educator)
- (Researcher)
Research
Experimental Design
This work was conducted in two randomized complete block experiments with four replicates in Minnesota over two years, 2021-2022. Field sites included the Minnesota Agricultural Experiment Station in St. Paul, MN (StP), and Big River Farm (BRF) in Marine on St. Croix, MN. BRF is part of The Food Group and provides land access and training in organic vegetable production and marketing to new immigrant and minority farmers. Farmer collaborators at BRF expressed interest in the study because of their observation that small management changes can dramatically change the efficacy of cover crops.
Experimental plots measured 0.93m2 in area. Cover crops (Table 1) were planted each spring (April-May) and were followed by a fall broccoli cash crop. The spring-planted cover crops were terminated mid-summer by mowing and incorporation with a walk-behind BCS tractor. Seeding rates for the cover crop treatments followed guidelines from seed suppliers. Primary tillage with a rototiller occurred each spring when soil moisture conditinos were suitable, typically mid-April. Secondary tillage with a field finisher occurred within a day of seeding. Primary and secondary tillage were repeated in July following cover crop termination. Broccoli seedlings were transplanted immediately following secondary tillage after cover crop termination. Broccoli was transplanted by hand into 1.8 m twin row beds with 76 cm between twins and in-row spacing of 46 cm.
Table 1. Legume and non-legume species included in the field design. Weed-free and weedy fallows served as control treatments to examine microbial processes in the absence of any cover crops.
Treatment | Cover crop species |
1 | Pisum sativum (Field pea) & Arrhenatherum elatius (Oat) |
2 | Trifolium incarnatum (Crimson clover) |
3 | Fagopyrum esculentum (Buckwheat) |
4 | Weed-free fallow |
5 | Weedy fallow |
Site Description
The StP site is located in Ramsey County, MN and has an average annual precipitation level of 828 mm. The soil type at this location is Waukegan silt loam. Mean monthly temperatures range from -10.5°C in January to 22.6°C in July.
Soil Sampling
To investigate the contribution of decomposing cover crop material to microbial N cycling processes, we collected bulk soil samples 0, 30, and 60 days after cover crop termination in 2021 and 2022. All soil sampling occurred during the transplanting and growth stages of the broccoli cash crop. Soils were collected to a depth of 10 cm using ascetic technique to prevent microbial cross-contamination between treatments. Briefly, ten soil cores were collected from each plot, avoiding sampling from within 30 cm of the plot border. Before collecting soil cores, surface plant residue was removed. Cores from the same plot were pooled in sterile plastic bags. In between sampling different plots, a 70% ethanol solution was used to clean and sterilize the soil probe. Bulk soil samples were immediately placed in a cooler at kept at 4C until processing. All time-sensitive microbial enzyme assays were performed within one week of the sampling date. Within one week, a portion of the bulk soil sample was set aside and stored at -20C until DNA extractions could be performed.
Nitrification Potential (NP)
Nitrification potential (NP) refers to the maximum rate of activity among nitrifying organisms under optimal conditions. Determining the NP of a soil community can give us useful information as to how a specific management strategy or crop species influences the potential rate at which nitrogen is cycled through soil by microbes. High rates of NP during moments of rapid crop growth and N uptake are favorable; during other times, NP should be managed to reduce N loss to the environment. To measure NP in summer cover crops, we used a modified protocol from Kandeler et al. (2011) in Methods of Soil Enzymology (ed. Richard P. Dick).
In brief, a NP solution was made that consists of potassium phosphate and ammonium sulfate which provides nitrifying microbial communities with the substrate required for their growth and activity. The solution was calibrated to the pH of the native soil, so that the enzymatic potential obtained from the assay is more reflective of a true potential. Within one week of sampling, 2.5g of fresh soil was mixed with 25 ml of the NP solution and placed on an orbital shaker at 180 rpm for 36 hours. Over the course of the 36 hour incubation, the soil slurry is sampled four times: 2 hours, 12 hours, 24 hours, and 36 hours. Finally, nitrate (NO3- ) concentration was determined using colorimetric methods. NP was calculated as the rate of NO3- production over the course of the incubation period.
Denitrification Enzyme Activity (DEA)
DEA is a soil incubation assay that is similar to NP in that it measures the maximum potential activity of denitrifying organisms in soil under optimal conditions. For denitrifiers, 'optimal' conditions refer to anaerobic or anoxic conditions and an adequate amount of substrate: glucose as a carbon source and nitrate. High rates of DEA are seldom favorable, since the end products of the microbial denitrification pathway are gaseous forms of nitrogen (N2 and N2O) that are lost from the system. To measure the extent to which different summer cover crop plantings impact DEA rates, we used a modified protocol from Tiedje (1994).
A DEA solution was prepared using 1mM glucose and 1mM KNO3- and adjusted to the average pH of the soil samples for each location. Prior to running the assay, we prepped 9 ml gas sample vials by sealing them with airtight septa. Within one week of sampling, 2.5g of fresh soil and 10 ml of solution were added to 160ml serum vials. Both serum vials and sample vials were evacuated using a vacuum pump (-26.5 psi) and subsequently re-pressurizing to 15 psi with pure N2. This evacuation and pressurization cycle was repeated three times. On the final cycle, the pressure was brought down to 0.1 psi after flushing with N2. The start time for the incubation was counted as the time that C2H2 (10ml) was added to the sample vials. C2H2 serves to inhibit the final conversion of N2O to N2 in the denitrification pathway so that only a single product is obtained from the incubation. Samples were incubated for 90 min, and 10ml samples of the vial headspace were collected at 0, 45, and 90 min. Gas samples were stored in the prepped 9ml sample vials until analysis on a GC/MS, typically within one day of running the assay. DEA is reported as the rate of N2O produced during the course of the incubation.
DNA Extraction and Sequencing
Soil was stored at -20C prior to extracting DNA. Total genomic DNA was extracted from 0.25g fresh soil using Qiagen DNEasy Powersoil Pro kit. Extracted soil was again stored at -20C until amplicon sequencing for 16S (bacteria) and ITS2 (fungi).
Extracted DNA was submitted to the University of Minnesota Genomics Center (UMNGC) for amplicon sequencing of the 16S rRNA and ITS2 regions to characterize bacterial and fungal communities, respectively. Briefly, the V4 region of the 16S rNRA gene and fungal ITS2 region between 5.8S and 23S rRNA gene were amplified with the Meta_V4_515F and Meta_V4_806R primer set (Gohl et al, 2016) and the 5.8SR_Nextera and ITS4_Nextera primer set (Castle et al, 2018), respectively. PCR products were subsequently purified and attached to dual index tags and Illumina sequencing adaptors as described previously (Gohl et al, 2016). Paired-end sequencing was completed using a MiSeq platform (Illumina) with V3 chemistry (300 bp read length).
We used mothur v1.48.0 to perform quality filtering and assembly of raw sequence reads (Schloss et al, 2009). Bacterial 16S reads were clustered into operational taxonomic units (OTUs) at 97% sequence similarity using the OptiCulust method in mothur (Westcott and Schloss, 2017). Bacterial OTUs were then aligned to the Silva 138.1 database for taxonomic classification. We used a similar OTU-based approach to process fungal ITS2 reads, but used a 95% threshold using the abundance-based greedy clustering method in mothur. Fungal OTUs were aligned to the UNIT v9.0 reference dataset for taxonomic classification.
Rarefaction was performed for 16S and ITS2 sequences at the level of 8,988 and 5,071, respectively. Quality-filtering of rare OTUs with a relative abundance of less than 0.01% was also performed for both datasets. Good's coverage at these rarefaction levels was 0.92 and 0.98 for 16S and ITS2, respectively, indicating that the vast majority of sequences were detected. To test the relationships between cover crops, N-cycling activity, and microbial communities, we conducted PERMANOVA analyses with adonis2, using Bray-Curtis distance matrices (computed using vegdist) from the vegan package in R. DESeq2 was used to identify individual taxa that were associated with cover crop treatments and levels of N-cycling activity.
Denitrification Enzyme Activity
On average, cover crop treatment did not influence denitrification enzyme activity (DEA) following spring-planted cover crop termination at either of the two study locations (p=0.06 in BRF and p=0.65 in StP). We also analyzed differences in DEA among cover crop treatments within each sampling time point for each location. There were no differences among any of the treatments at any time point at BRF. In St Paul, the weed free control plot demonstrated lower DEA (p=0.05) than the pea/oat cover crop mixture at the first time point only (at cover crop termination; Fig 1). Despite the nonsignificant results, the weed-free and buckwheat treatments tended to have lower DEA overall in both locations.
DEA was more strongly influenced by soil moisture rather than cover crop effects. Average DEA varied significantly between each sampling time point for both BRF and St Paul (p<0.05) in both years. At both locations, DEA was greatest at the second time point (30 days post termination) and then decreased at the third time point (60 days post termination). DEA was lower at the time of cover crop termination compared to the other time points at both locations. The second sampling time point occurred in August, when soil and moisture temperatures were at their highest levels of the season (Fig 1). Warm temperatures and high soil moisture are both factors that are known to increase microbial N cycling activity. The relationship between soil moisture and DEA was stronger at StP than at BRF (Fig 2B).
These results concur with our expectation that DEA would increase during the experiment as cover crop residues decomposed following termination. The initial time point at cover crop termination is a close representation of living cover effects on denitrifying microbial activity. BRF had lower soil organic matter then St Paul, which could account for non-significant treatment differences if carbon availability was a limiting factor for microbial growth. As expected in St Paul, the weed-free treatment exhibited lower DEA than the pea/oat mixture, which had the highest DEA. Notably, the legume-only treatment, crimson clover, did not have higher DEA than any of the other legume or non-legume treatments. These results suggest that crimson clover may be a promising summer cover crop to fill both nitrogen fertility and retention goals. Crimson clover contributes organic nitrogen to the soil via biological nitrogen fixation, but does not appear to stimulate denitrifying enzyme activity that would lead to N loss during residue decomposition.
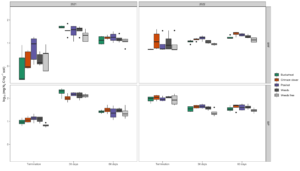
Nitrification Potential
Trends in nitrification potential (NP) over the course of cover crop decomposition were similar to the DEA results. This was expected, given that the end product of the nitrification pathway, NO3-, is also the starting substrate for the denitrification pathway. Because there is facilitation of nitrogen products between nitrifying and denitrifying microorganisms, we expected NP and DEA results to be highly similar. At both BRF and StP, there was a strong correlation between nitrification and denitrification enzyme activity across all timepoints (Fig 2A). As with DEA, the relationship between soil moisture and NP was stronger at StP than at BRF.
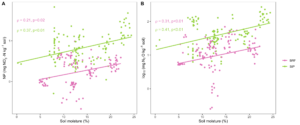
Overall, NP was affected by cover crop treatment across all sampling timepoints in each location (p<0.001 in BRF and p=0.02 in StP). In BRF, NP in the weed-free control plot was significantly lower than all other treatments by more than a factor of two. In StP, however, the weed-free control did not differ from the pea/oat or crimson clover treatments but had significantly lower NP than both buckwheat and the weedy fallow. This result was not surprising, since weed-free plots have lower plant-derived nitrogen inputs compared to plots with living cover. During the first year in BRF, we obtained negative NP for the weed-free treatment (Fig 3), suggesting potential immobilization of available soil nitrogen by microorganisms. By year two, NP values had increased in BRF but decreased in StP. However, treatment effects remained the same.
As cover crop and weed residues decomposed during the summer broccoli cash crop, we observed significant increases in average NP for all treatments in BRF (Fig 3). The highest rates of NP occurred 30 days after cover crop termination, while the lowest rates of NP occurred at the last time point, 60 days after termination in both years. Even while NP and DEA are correlated due to the metabolic interdependence of the microorganisms involved, the differential effect of season on NP compared to DEA suggests that the nitrifying community responds differently to environmental factors. In particular, nitrification processes seem to be more plant-driven than denitrification. However, DEA values were greater on average than NP values, indicating that gaseous soil emissions likely account for a larger share of N loss in these organic vegetable systems than nitrification. Nitrification is an aerobic process, so we would expect NP to exhibit a smaller response to increases in soil moisture as compared to DEA. Ongoing analysis involving our soil moisture and temperature probes will help to clarify specific environmental drivers on NP. In StP, the highest rates of NP (time point 2/ 60 days post-termination) coincided with an active period of broccoli growth. This implies that all cover crop treatments performed equally well in this location in supplying N during a critical period of cash crop growth.
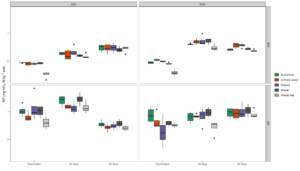
Microbial Community Dynamics with Respect to Cover Crop Treatment and N-Cycling Activity
Across all samples, bacterial 16S rRNA community composition was strongly influenced by site, soil collection date, and cover crop treatment. After accounting for the effects of location and collection date and their interaction, cover crop species accounted for 2.1% of observed variation in community structure (PERMANOVA, p<0.01). Within site, cover crop treatment accounted for even greater proportions of 16S community structure. Cover crop treatment explained 7.8 % (p=0.03) and 9.4% of variation in 16S community structure and in BRF and StP, respectively.
As expected, community structure was also strongly tied to measures of microbial-driven N-cycling activity (Fig. 4). After accounting for location, cover crop treatment, and sample collection date, both NP and DEA were significant in accounting for total variation in 16S community structure across all samples. Globally, NP explained 0.7% (p=0.06) and DEA explained 2.2% (p<0.01) of variation in 16S community structure across sites. Within sites, DEA tended to be more strongly related to community structure than NP. In BRF, NP accounted for 2.7% (p=0.07) and DEA accounted for 5.7% (p<0.01) of variation in 16S community structure. Similar results were obtained in StP, wherein NP explained 3.6% (p=0.02) and DEA explained 4.9% (p<0.01) of variation in 16S community structure after accounting for cover crop treatment and sample collection date. Bacterial communities were thus important mediators of nitrification and denitrification activity in bulk soils, and were also strongly influenced by summer cover crop species identity. These findings corroborate our hypothesis that cover crop species selection can indirectly govern N loss in agroecosystems by modulating bacterial community composition.
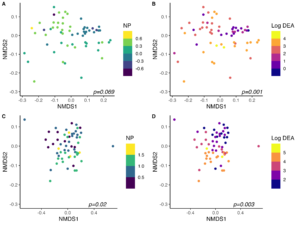
While bacterial communities were strongly linked to rates of nitrification and denitrification potential activity, fungal community structure was related solely to denitrification activity after accounting for the interactive effects of cover crop species, collection date, and site across all samples. After accounting for site, cover crop treatment, sample collection date, and their interactions, NP did not account for a significant amount of variation in fungal ITS2 community structure (PERMANOVA p=0.12). However, DEA was significant in explaining 2.2% of variation in community structure after accounting for other variables (p=0.001) across all samples. These results concur with our understanding that fungi contribute substantially to denitrification in soils, while their role in mediating nitrification is less well defined and potentially more phylogenetically constrained.
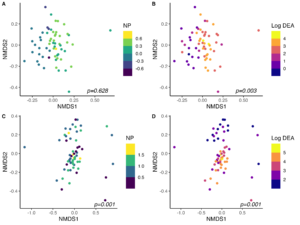
Within site, however, fungal community structure was significantly related to NP in Saint Paul, where NP explained 5.1% of variation in fungal community structure (p=0.001). DEA was highly related to fungal community structure in both locations, accounting for 6.2% of variation in community structure in Saint Paul (p=0.001) and 3.4% of variation in fungal community structure in BRF (p=0.003).
Differential abundance of microbial taxa
Cover crop species treatments resulted in 41 differentially abundant bacterial OTUs (Bonferroni adj. p < 0.05) in bulk soil. We did not identify any fungal OTUs whose relative abundance was associated with cover crop treatment relative to the weed-free control. Eleven bacterial OTUs, which belonged to the genera Tumebacillus, Nakamurella, and unidentified Bacillales were either differentially increased or decreased across all treatments (buckwheat, crimson clover, pea/oat, and weedy fallow) relative to the weed-free control. Of these eleven OTUs, four included OTUs belonging to the genus Nakamurella, which had significantly enhanced abundance across all cover crop treatments relative to the weed-free control plot. On the other hand, the remaining seven OTUs that were classified as Tumebacillus and unidentified Bacillales were associated with decreased abundances across cover crop treatments relative to the weed-free control. These results imply that summer cover crop species are capable of modulating the abundance of specific bacterial taxa in the bulk soil over the course of their relatively short growth period.
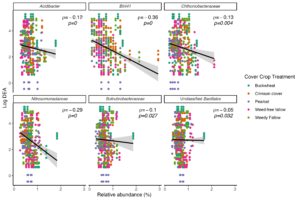
In order to identify whether the bacterial taxa that responded to cover crop presence in bulk soil were associated with reduced potential N loss, we performed Spearman's correlation tests between OTU relative abundance and potential N-cycling activity. We identified six differentially abundant taxa that were significantly negatively associated with DEA (Fig. 6) and three taxa that were significantly negatively associated with NP (Fig. 7). Crimson clover was associated with four of the seven OTUs whose relative abundance was negatively correlated with DEA, and all three of the OTUs that were negatively correlated with NP. Relative to the weed-free control, crimson clover treatments resulted in a 1.5-fold increase in the abundance of OTU705, which belongs to a family-level group Blrii41. While this bacterial family has no documented roles in N cycling, OTU705 was negatively correlated to both NP and DEA, suggesting that it may be a powerful competitor with nitrifiers and denitrifiers for N use.
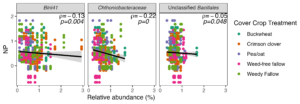
Ultimately, our results indicate that summer cover crops influence rates of potential N loss in agroecosystems. Despite their limited window of growth between spring and fall cash crops, summer cover crops can positively or negatively impact the abundance of select bacterial taxa in the bulk soil community. The fact that cover crops were not able to affect the abundance of individual fungal taxa concurs with our established understanding of fungal life history strategies that involve longer generation times than bacteria, whose abundance is more likely to respond dynamically to nutrient inputs or plant signals. We also found that while bacterial community structure was highly related to both nitrification and denitrification potential activity, fungal community structure was only consistently related to denitrification potential activity. Crimson clover treatments resulted in the greatest number of differentially abundant bacterial taxa that were negatively correlated with potential rates of nitrification and denitrification. Taken together, our results suggest that crimson clover may be a 'best bet' cover crop to maximize organic N inputs while limiting microbial sources of N loss via nitrification or denitrification on a field scale.
Educational & Outreach Activities
Participation Summary:
1. Summer research symposium at the University of Minnesota
In August 2021, we presented a poster at a University of Minnesota research forum that attracted educators and students from diverse disciplines. We reached an estimated number of 30 students and educators at this event. The topic of the presentation was "Microbial denitrification activity in summer cover crop mixtures".
2. ASA/CSSA/SSSA Annual Meeting
We presented complete results from the first field season of this study at the annual Agronomy Society of America/Crop Science Society of America/Soil Science Society of America meeting in November 2021 in Salt Lake City, Utah. The title of our poster presentation was "Maximizing summer cover crop conservation benefits for improved vegetable production". We engaged with an estimated number of 50 scientists, students, educators, and extensionists at this event.
3. Minnesota State Fair Outreach and Education Booth
In August 2021, we facilitated a 4-hour long outreach and extension booth in the Horticulture Building at the Minnesota State fair. We interacted with an estimated number of 50 members of the general public, which included students, homeowners, and farmers. At this event, we conducted a hands-on experiment to demonstrate the role of microorganisms in providing soil nutrients and contributing to health soil structure. We also displayed different cover crop options, and provided advice on when/why to apply microbial inoculants.
4. MOSES Organic Farming Conference, 2022
In February 2021, we presented a poster at the Marbleseed (formerly MOSES) Organic Conferene in LaCross, WI. We interacted with an estimaed number of 30 conference attendees, 10 of whom were farmers. Through these interactions, we were successful in educating farmers and the general public about the effect of cover crops on microbial N transformations in soil.
5. Field Day: Emerging Farmers Conference, 2022
We held a field day for 100 emerging and minority farmers in November 2022 at the Good Acre in St Paul, MN. At this event, we discussed microbial N cycling functions in soil. Due to audience interest, we focused on a discussion of N contributions from legume cover crops and compared this fertility source to other organic amendments such as compost. We created and distributed a handout that illustrated the role of soil bacteria in contributing N to organic soils.
6. Minnesota Organic Conference, 2023
Our final outreach event was the 2023 Minnesota Organic Conference in St Cloud, MN in January. We presented an hour-long talk for 50 attendees that included farmers and industry professionals. Our talk included live demonstrations to highlight microbial diversity and function in soil. We paid particular attention to microbial N cycling and discussed practical steps that farmers can take to cultivate beneficial, diverse microbial communities in their soil. At this event, we also distributed a survey to understand how farmers rate microbial function in relation to other ecosystem services from cover crops.
Project Outcomes
Spring-planted cover crops provide organic vegetable farmers with an opportunity to introduce a diversity of ecosystem services into their summer cash crop rotations. In the short growing season of the upper Midwest, identifying optimal cover crop mixtures that maximize services such as soil fertility within a limited window of time remains a challenge. In particular, greater knowledge of how cover crops interact with soil microbiota will help to inform growers with a balanced perspective of potential nitrogen fertility and nitrogen retention services. Here, we assessed how cover crop residue decomposition affects nitrogen cycling microbial activity across three treatments that were identified by our farmer collaborators: a non-legume (buckwheat), a legume only (crimson clover), and a grass/legume mixture (pea/oat). Our preliminary findings suggest that crimson clover may offer the best balance of N retention and provision services because it did not stimulate potential N loss due to denitrifying soil activity during the warmest and wettest period of residue decomposition in mid-August. Crimson clover was also able to influence the relative abundance of several bacterial OTUs which were negatively associated with both NP and DEA.
This project has helped to improve our awareness of how summer cover crop decomposition processes impact soil microbial activity. We were surprised to find few differences between legume and non-legume cover crop treatments. These preliminary results could suggest that legume cover crops provide a longer-term, stable source of organic nitrogen for cash crop growth without enriching microbes that would rapidly metabolize nitrogen inputs, leading to its loss. In particular, crimson clover appears promising because it did not stimulate nitrifying or denitrifying activity relative to other treatments, including controls. Crimson clover has also performed well in recruiting beneficial insects, which is an emerging finding from the larger SARE grant to which this student fellowship contributes. This work allows us to holistically consider multiple ecosystem services from summer cover crops and provide recommendations to organic growers.