Final report for GNE16-131
Project Information
A growing number of farmers in the United States, especially the Northeast, have adopted sustainable management practices including reduced tillage and cover cropping. In the absence of tillage, growers must rely on a diverse and active decomposer community to break down residues and recycle nutrients. Insufficient residue break down may exacerbate pest problems, e.g. slugs, and reduce stand counts in subsequent growing seasons. Decomposer diversity and activity may be reduced by prophylactic pesticide use, which may inadvertently slow residue break down.
My research goal for this proposed research was to investigate the effect of prophylactic pesticide use on arthropod-mediated decomposition in Northeastern soybean and maize production. I focused on the pyrethroid lambda-cyhalothrin and neonicotinoid seed coats (imidacloprid and clothianidin) due to their high prevalence but disputed pest control value in the Northeast.
I designed a three-year field experiment in maize and soybeans plots under no-till, cover-crop management. A third of my plots were planted with neonicotinoid coated seeds, a third received an early-season foliar spray of a pyrethroid (lambda-cyhalothrin), and a third received no insecticide treatment. I measured decomposer abundance and activity using a combination of pitfall traps and litterbags.
The macro-decomposer community in soybean and corn fields is dominated by a single millipede species, Oxidus gracilis, which appears to have variable population responses to pyrethroid exposure. While specific decomposer populations were reduced by both pesticide treatments, the pyrethroid had greater negative effects on the dominant decomposer group (soil mites). As a result, decomposition was not affected by neonicotinoid seed coats, but was slowed under pyrethroid application. However, while statistically significant, this effect on decomposition was small and therefore unlikely to have a noticeable effect on residue break down.
Despite these negative effects on decomposers and decomposition rate, neither insecticide increased crop yield. At least in the Northeast, pest insect populations usually fall below treatment thresholds, and excessive insecticide application may reduce the effectiveness of sustainable management practices. Overall, these results provide additional justification for growers to reduce unneeded insecticide applications in field crops.
Project Objectives:
I had four main objectives:
Objective 1: Define the surface-active arthropod decomposer communities in conventional soybean and corn fields of the Northeastern U.S., and Pennsylvania in particular.
Objective 2: Determine the influence of macro-decomposers on decomposition characteristics, including residue breakdown rate and nutrient mineralization.
Objective 3: Determine the acute sensitivity of representative macro-decomposers to the pyrethroid lambda- cyhalothrin and the neonicotinoids clothianidin and imidacloprid.
Objective 4: Assess how prophylactic insecticide use (neonicotinoid seed coats and post-planting pyrethroid applications) affects surface-active arthropod decomposers and decomposition.
A growing number of farmers in the United States, especially the Northeast, have adopted sustainable management practices including reduced tillage and cover cropping (Baumgart-Getz, Prokopy, Flores 2912; Derpsch, Friedrich, Kassam, Li 2010; Kaye and Quemada 2017; Roesch-McNally et al 2017). These practices are primarily adopted for erosion control, improving soil quality, and nutrient management (Baumhardt, Stewart, Sainju 2015; Jabbour, Pisani-Gareau, Smith, Mullen, Barbercheck 2015; Schipanski et al. 2014; Schmitz, Buchkowski, Smith, Telthorst, Rosenblatt 2017; Varela, Barraco, Gili, Taboada, Rubio 2017); however, these sustainable management practices also influence in-field invertebrate diversity and activity, which can alter pest communities and promote biocontrol of pests
With cover crops and reduced tillage, is becomes more challenging to manage residues – instead of relying on mechanical incorporation and breakdown of crop and cover crop residues, reduced tillage systems have to rely on the biological activity of decomposers (Hendrix, Parmelee, Crossley, Coleman, Odum , Groffman 1986; House and Parmelee 1985; House and Stinner 1987; Ossola, Hahs, Nash, Livesley 2016). To efficiently break down residues and recycle nutrients in the absence of tillage, the decomposer community must be diverse and active, with healthy populations of macroinvertebrates (earthworms and millipedes), mesofauna (springtails, mites, and potworms), and microfauna (protists, bacteria, and fungi) (Donker, Eijsackers, Heimbach 1994; Grandy, Wieder, Wickings, Kyker-Snowman 2016).
Decomposer diversity and activity may be reduced by pesticide use. This especially concerning if pesticides are being used prophylactically, e.g. when growers apply insecticides without scouting and when they deploy insecticide seed coats. If insecticide seed coats affect decomposers, these treatments may inadvertently increase the persistence of surface residues and alter nutrient release from residues (Donker, Eijsackers, Heimbach 1994; Zaller et al. 2016). While high cover crop residue levels before the growing season can help maintain natural enemy populations (Jabbour, Pisani-Gareau, Smith, Mullen, Barbercheck 2015; Schipanski et al. 2014), slow residue breakdown may exacerbate pest problems, e.g. slugs, which benefit from high residue retention (Le Gall and Tooker 2017; Douglas 2012; Douglas, Rohr, Tooker 2015).
My research goal for this proposed research was to investigate the effect of prophylactic pesticide use on arthropod-mediated decomposition in field crop systems. I focused on fungicide and neonicotinoid seed coats due to their ubiquity in soybeans and maize produced in the United States (Douglas and Tooker 2015), despite inconsistent yield benefits (Douglas and Tooker 2015; Krupke and Alford et al 2017; North et al. 2016; North et al. 2017). This research is important for sustainable agriculture in the Northeast, as sustainable pest management methods should minimize detrimental effects on other managed aspects of farming (e.g. pollination, nutrient cycling, and soil health).
Cooperators
- (Educator and Researcher)
Research
Materials and methods:
Field Management (objectives 1, 2, & 4)
In June 2016 at Penn State’s Entomology Research Farm, I planted soybeans (South Field – 60 x 60 ft plots) and field corn (North Field – 50 x 70 ft plots) in two no-till fields (Figure 1 and Table 1). Plots either received no insecticide (Control), received a post-plant pyrethroid spray (Pyrethroid), or were planted with neonicotinoid coated seeds (Seed Coat). Both soybeans and maize were planted in 30 inch rows.
After soybeans and corn grain harvest in the fall, we planted rye as a cover crop in all of the plots. The following spring, we used Glyphosate and 2-4,D to burn down the cover crop before planting.
In 2017, we rotated corn and soybeans but kept plot treatments otherwise consistent with 2016. Like in 2016, we planted a rye cover crop in the fall which was burned down before planting in 2018.
In 2018, we returned the South Field (60 x 60 ft plots) to soybeans and the North Field (50 x 70 ft plots) to field corn. However, due to persistent flooding and planting errors, sampling in North Field was incomplete for 2018.
Sampling and Identification of Surface-Active Arthropods (objectives 1 & 4)
Macro-decomposers
I used pitfall traps to sample surface-active macroarthropods (figure 2). Pitfall traps comprised clear deli containers (16 oz) set into the soil, each with 50-70 mL of 50% propylene glycol. Plastic plates were used as covers to protect the pitfalls from rain and to keep out vertebrates. In 2016, I opened two pitfall traps in each plot for 48 hours – I did this on July 5th, July 19th, and August 30th. In 2017, pitfall traps were opened for 72 hours on July 20th (North Field, soybean plots), July 27th (South Field, maize plots), and Oct 14th (all plots). In 2018, pitfall traps were opened for 72 hours on July 27th and Oct 12th (South Field only). All specimens collected from pitfall traps were transferred to 80% ethanol to be counted, identified, and archived.
Mesofauna decomposers
Initially I designed this project to focus on just macro-decomposers; however, I decided to expanded my study to include decomposer mesofauna which I could extract from the litterbags I deployed for objectives 2 & 4. To extract mesofauna, I placed litterbags on Berlese Funnels for 72 hours after collection (figure 2). Briefly, a Berlese Funnel is a large metal funnel (12” diameter) suspended under a 25 watt incandescent light bulb. At the base of the funnel is a cup with 80% ethanol. Soil organisms are driven downward by head and light, so they move down the funnel and eventually fall into the cup of ethanol. All specimens collected from litterbags were stored in 80% ethanol until they could be identified and counted. For each sample, mesofauna were identified and counted under a microscope; from here, specimens were transferred to glycerol on concave glass slides (one slides per sample). I took pictures of each slide for reference, then returned specimens to 80% alcohol to be archived.
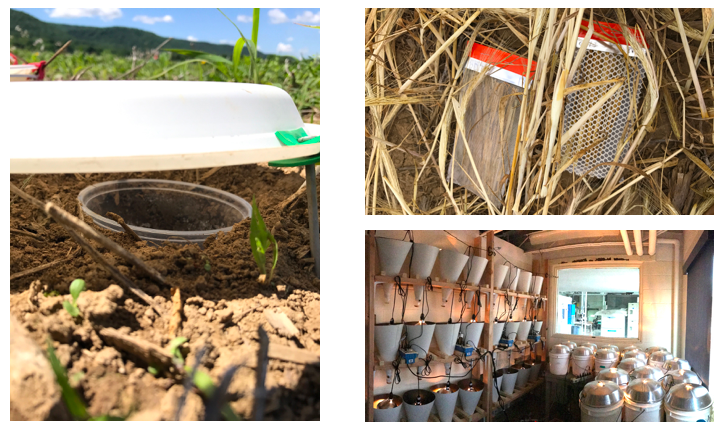
Litterbag Experiments (objectives 2 & 4)
I used litterbags to determine the influence of my pesticide treatments on decomposition rate. I based my method on the recommendations and guidelines developed at the Effects of Plant Protection Products on Functional Endpoints in Soil (EPFES) Workshop held in 2002 (Römbke et al, 2003). As I proposed, I placed pairs of litterbags in each plot; each pair of bags included a macro-decomposers-exclusion litter bag constructed from 1 mm polyester mesh and a macro-invertebrate-permitting bag with a 5 mm polyester mesh top (Figure 2). The litterbags were 10 cm by 20 cm flat rectangles containing either 3.90- 4.10 grams of rye straw (bags deployed in 2016) or 4.18- 4.30 grams of wheat straw (bags deployed in 2017 and 2018). While I originally had planned to use maize and soy litter in these bags, I decided to use straw instead; this eliminated the complications that could arise from using different litter types/qualities. As I used a rye cover crop, the decomposer community should have been ‘familiar’ with straw-like residue. Additionally, rye and wheat straw are standard litter for decomposition studies.
Litterbag Placement and Collection
I placed batches of litterbags in both spring and fall, to correspond to the times during the year where residues/litter deposition is highest (spring = cover crop termination, fall = crop residue post-harvest). In total, I placed five batches of litterbags; three in June right after planting, and two in November after harvest:
- In June 2016, I placed 300 pairs of fine and coarse-mesh litterbags (10 pairs per plot); subsets of the bags were collected in June, August, and November of 2016 as well as March, May, and July of 2017.
- In November 2016, I placed 270 pairs of bags (9 pairs per plot); subsets were collected in November 2016 as well as March, May, July, August, September, and November of 2017.
- In June 2017, I placed 180 pairs of bags (6 pairs per plot); subsets were collected in July, August, September, and November of 2017, as well as March and May of 2018.
- In November 2017, I placed 150 pairs of bags (5 pairs per plot); subsets were collected in March, May, July, August and October of 2018. Note: no bags from the flooded North Field were collected in July or October.
- In June 2018, I placed 90 pairs of bags (3 pairs per plot); subsets were collected in July, August, and October of 2018. Note: no bags from the flooded North Field were collected in July or October.
In total, I deployed 1,980 litterbags. 1,704 bags were used for decomposition analysis (this excludes bags collected immediately after placement in 2016 – to determine if any litter loss occurred during bag handling – and bags not collected in the flooded North Field during 2018).
In June 2016, pairs of bags were placed randomly in the field, however it was challenging to find bag pairs later in the season. For all of the following batches, I grouped the litterbags around the central two rows in each plot. Litter bags remained in place during most management activities (herbicide and fertilizer applications, corn grain harvest), but during planting and soybean harvest they were temporarily removed, held in individual plastic bags, stored at 4˚C, then replaced to their original field locations.
After extracting arthropods from litterbags (see Sampling and Identification of Surface-Active Arthropods), all litterbags were carefully rinsed then oven-dried at 55˚C. After drying, I carefully removed and weighed the remaining straw. Due to high soil contamination, replicate straw samples were pooled and sent to Dairy One (Ithaca, NY, USA) to determine ash-free dry weight (via combustion).
Nutrient Mineralization
Initially I had proposed analyzing the soil directly beneath each litterbag to assess nutrient mineralization under each treatment and litterbag mesh size. Due to logistical challenges and advice from my PhD committee, I decided to instead analyze litter chemistry over time; a subset of the litterbag samples from the South field were sent to Dairy One for protein and lignin analysis. Additionally, I sampled soil in May 2017 and November 2018, but on a plot-by-plot basis in the South Field as opposed to underneath each litterbag. Soil samples were analyzed for organic matter content, pH, cation exchange capacity, phosphorus, magnesium, potassium, calcium, sulfur, copper, and zinc.
Toxicity Assays (objective 3)
Initially, I had proposed the following:
In late spring and early summer, I will hand-collect and use dry pitfall traps to collect common macro-decomposers. Macro-decomposers will be housed in species-specific colonies and fed yeast.
For all species, I will run soil-based contact exposure assays with lambda-cyhalothrin, imidacloprid, and clothianidin. Individuals will be placed in new condiment cups with neonicotinoid-dosed soil. Target doses will be 1, 10, 100, 1000, 10,000 ppb, and a control. Toxic response and mortality will be assessed after 4 hours, 24 hours, and 7 days. Mortality will be determined as no movement stimulated by being flipped over with forceps. Toxic response will be determined as reduced movement or twitching. From these response, I will calculate approximate TC50s, LC50s, and LOECs. If time permits, and if I have enough specimens, I will repeat these assays at a finer resolution and multiple times to refine toxicity estimates.
However, I have run into challenges while trying to maintain lab colonies of macro-decomposers and running these assays. I had planned to keep colonies into the winter to avoid running assays during the busy field season, however this hasn’t been possible due to the biology (an annual life cycle) of the most common millipede species (Oxidus gracilis and Cylindroiulus caeruleocinctus) and the low density of isopods. I still plan to run standardized, acute dose-response assays on Oxidus gracilis, Cylindroiulus caeruleocinctus, and isopods.
Statistical Methods (all objectives)
All statistical analyses were performed in RStudio (version 1.1.463, running R version 3.5.1). Where appropriate, data were tested for normality and equal variance. Statistical methods included paired student t-tests (t.test), anova (aov), manova (manova), linear regression (lmer), and general linear mixed effect models (glmer and glmer.nb). Methods were chosen based on the type and fit of data. Error bars represent ± standard error, unless otherwise noted.
For the litterbag study, statistical analyses were performed on decomposition rate constants, k. These constants were derived by fitting the data to a first-order decomposition model, Xt/X0 = e-tk, where X0 is the initial amount of litter, Xt is the amount of litter left at time t, t is time in days, and k is the decomposition rate constant.
Objective 1 Results and Discussion:
Macro-decomposers
In Pennsylvania, the surface-active macro-decomposer community in soybean and corn fields was dominated by a single millipede species, Oxidus gracilis (figure 3). As this macro-decomposer community is virtually monospecific, any management practice or pesticide application that affects Oxidus gracilis will dramatic alter macro-decomposer contributions to decomposition. The remaining surface-active macro-decomposers were non-native Julidae millipedes (mostly Cylindroiulus caeruleocinctus) and isopods. I observed considerable evidence of earthworm activity (castings, tunnels), however pitfall traps are not effect for capturing earthworms; I hope to quantitatively measure earthworm activity/density in follow-up studies.
Despite a diversity of native macro-decomposers in Pennsylvania, the macro-decomposers in soybean and corn fields were nearly all non-native. This compares to the arthropod pests and predators in these fields, which are often non-native as well. It is likely that non-native/introduced species are better suited for these frequently disturbed, artificial habitats (Pearsons, Tooker, 2017).
Mesofauna decomposers
In addition to macro-decomposers, I identified mesofauna decomposers extracted from the litterbags used for objectives 2 & 4. The majority of mesofauna were either soil mites or collembolans (figure 3). Mites, especially oribatid mites, reach high densities throughout the season. Collembolans were the second most abundant group of mesofauna, but they were over five times less abundant than mites. Most of the collembolans extracted were from the order Entomobryomorpha, although there were many from Symphypleona at certain timepoints, and some from Poduromorpha. While macro-decomposers are more conspicuous, mesofauna decomposers may contribute more to decomposition due to their higher abundance.
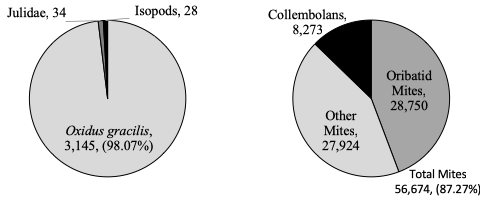
Objective 2 Results and Discussion:
Collembola were significantly more abundant in coarse mesh litterbags (t-test, mean difference between paired coarse and fine litterbags, 5.98 ± 2.83, P < 0.0001), however no other group of decomposers were affected by mesh size (Figure 4). Even millipedes were equal in fine and coarse mesh litterbags, despite the fine mesh bag having been designed to exclude macro-decomposers. Millipedes were rare in litterbags, and those that were present were usually juveniles small enough to pass through the 1 mm fine mesh. Additionally, densities of predators (beetles, spiders, and centipedes) did not differ between mesh sizes, so it is unlikely the fine mesh bags protected smaller decomposers from potential predation (Figure 4).
Overall, these results suggest the use of coarse and fine mesh litterbags to include/exclude macroinvertebrates may not be robust enough to demonstrate the effect of macro-decomposers on decomposition; even if macroinvertebrates can be successfully excluded from fine mesh bags, this would exclude not only macro-decomposers, but also invertebrate predators. Top-down trophic forces could potentially be misconstrued as effects of macro-decomposers. I plan to do a more controlled follow-up experiment using microcosms and introducing macro-decomposers, instead of trying to exclude them in a field context.
Decomposition and Macro-invertebrate Exclusion
Preliminary results suggested decomposition was faster in coarse mesh bags, especially the breakdown of more recalcitrant compounds (e.g. lignin). However, the overall results of this study don’t follow those preliminary results. Mesh size did not significantly affect decomposition rate in either the South or North field (linear mixed effects model; South Field: F = 0.7111, d.f. = 1054, P = 0.3993; North Field: F = 0.5099, d.f. = 571.09, P = 0.4755). Additionally, mesh size did not affect protein:lignin ratios (paired t-test, difference between paired coarse and fine litterbags, 95% CI = (– 0.0093, 0.035), P = 0.2479). As there was no significant effect of mesh size on the abundance of decomposers, these results are not unexpected. Similar results have been found in forest studies when juvenile millipede and isopods can enter finer mesh litterbags (Anderson 1973). Decomposition is only faster in coarse mesh litterbags when macro-decomposers are abundant and successfully excluded from fine mesh litterbags.
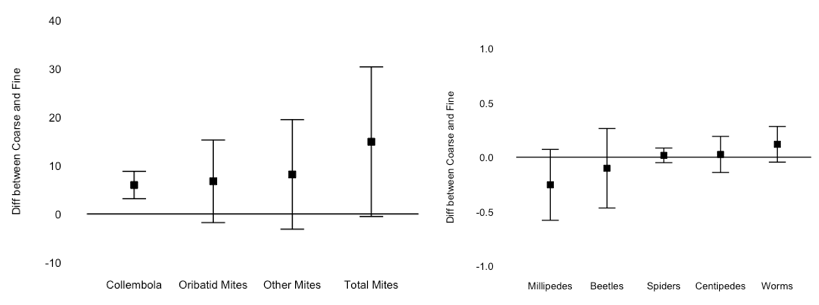
Objective 3 Results and Discussion:
I did run a series of soil-exposure toxicity assays with O. gracilis, however the neonicotinoid active ingredients did not fully dissolve in the water used to dose soil. Assays will be re-run using a similar method I developed to toxicity assays on carabid beetles (using acetone).
Field and lab assays have shown toxic effects of neonicotinoid seed coats on carabid beetles (Mullin, Saunders, Leslie, Biddinger, Fleischer 2005; Leslie, Biddinger, Mullin, Fleischer 2009), namely lethal effects and feeding inhibition. The assays I ran with two carabid species (Harpalus pensylvanicus and Pterostichus melanarius) supported these results (figure 5), and I am optimistic I will be able to continue these assays and successfully run similar assays with O. gracilis this following spring.
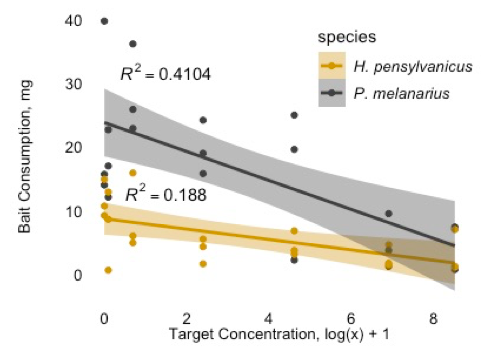
Objective 4 Results and Discussion:
Because the North Field was consistently wetter and often covered in inches of standing water, results from the North Field less consistent than results from the South Field. Thus for the following, results from the South and North field have been separated.
Macro-decomposers: Oxidus gracilis
South Field. As noted in the results for objective 1, the decomposer community was dominated by the millipede Oxidus gracilis. Compared to controls, O. gracilis activity-density was unaffected by the use of neonicotinoid seed coats, but did reduce by nearly 60% after post-planting pyrethroid applications in 2017 and 2018 (negative binomial generalized linear model; d.f. = 70, P= 0.0304). (Figure 6). These results suggest that at least one millipede species, O. gracilis, is not sensitive to neonicotinoids at field concentrations. No studies have been published regarding millipede sensitivity to neonicotinoids, so I cannot speculate whether this can be generalized to other millipede species. This lack of data highlights the need for the neonicotinoid-based toxicity assays I proposed for objective 3. However, millipedes, including O. gracilis, have been show to be sensitive to multiple pyrethroids (Francisco and Fontanetti 2015). The reduction of O. gracilis activity-density in response to λ-cyhalothrin support this generalization. In systems where millipedes are key decomposers, pyrethroids will likely slow decomposition.
North Field. Overall O. gracilis activity-density was much higher in the North field than the South fields. Additionally, O. gracilis activity-density actually increased by nearly 50% in response to pyrethroid exposure in July of 2016 and 2017 (negative binomial generalized linear model; d.f. = 34, P = 0.0016) (Figure 6). This increase in activity-density seems contrary to both the results in the South field and the results found with other millipede species and pyrethroids. Increased O. gracilis capture may be a result of sublethal effects; O. gracilis convulses and loses coordination when exposed to sub-lethal levels of cypermethrin (Gromysz-Kalkowska and Szubartowska 1994). Under sub-lethal exposure, convulsing and uncoordinated O. gracilis may be more likely to fall into pitfall traps. While millipedes in the South Field likely encountered lethal doses to λ-cyhalothrin, the wetter and weedier conditions in the North Field may have resulted in sub-lethal λ-cyhalothrin exposure.
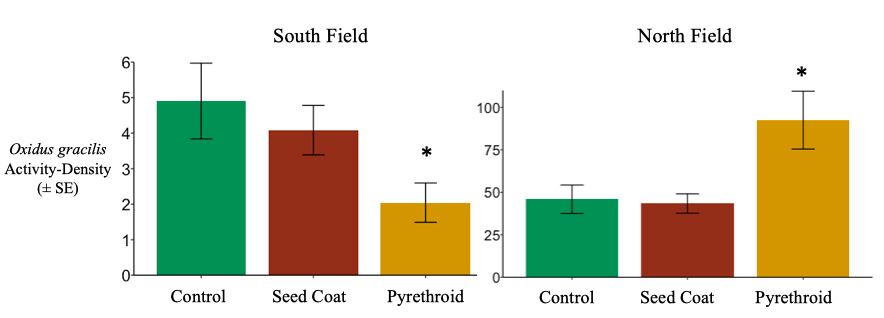
Mesofauna
South Field. Total soil mite densities in litterbags were unaffected by neonicotinoid seed coats, yet were reduced by nearly 25% after post-planting pyrethroid applications (negative binomial generalized linear mixed effect model; χ2 =10.911, d.f. = 2, P = 0.004273). (Figure 7).
Studies have shown phytophagous mite populations to increase in response to neonicotinoids (Pisa et al. 2014), and one species of oribatid mite, Oppia nitens, was quite insensitive to imidacloprid in a laboratory assay (Lima e Silva et al. 2017). Oribatid mites have been shown to reduce surface activity under imidacloprid application (El-Naggar and Zidan 2013). However, certain pyrethroids, including cyhalothrin, have high enough acaricidal activity to be for control of pest mites (O’Reilly et al. 2013). Our observations support these previous findings.
Collembolan densities in litterbags were reduced by the neonicotinoid seed coat by about 25%, although this result is only marginally statistically significant; the pyrethroid reduced collembolan densities by a similar margin (25%), but this was not statistically significant (negative binomial generalized linear mixed effect model; χ2 =5.5155, d.f. = 2, P = 0.06343). (Figure 8). Folsomia candida has been show to be sensitive to imidacloprid but not thiamethoxam in toxicity assays (Lima e Silva et al. 2017; Seres et al. 2016), however collembola abundance has been shown to increased in soil in response to imidacloprid seed coats (El-Naggar and Zidan 2013). Different collembola species are found in surface litter than within the soil, so it is likely that the species I was extracting from litterbags have different physiology than those extracted from soil. The species I was capturing at the soil surface may be more sensitive than those in the soil. It is also possible that collembola are congregating in the soil when exposed to neonicotinoid seed coats; they may be drive into the soil away from the litter, or they may not be leaving the soil to feed in the litter (either due to sublethal effects or avoidance behavior). These hypotheses could be tested by sampling both litter and soil, in addition to studying collembola at finer taxonomic levels.
North Field. Soil mite densities did not differ across treatments (negative binomial generalized linear mixed effect model; χ2 = 0.4038, d.f. = 2, P = 0.8172). Collembolan densities also did not differ across treatments (negative binomial generalized linear mixed effect model; χ2 = 1.8335, d.f. = 2, P = 0.3998). Overall, mesofauna densities were lower in samples taken from the North Field. The wet and often flooded conditions in the North Field likely overshadowed any effect of pesticide treatment on mesofauna.
Decomposition rate, k
South Field. Decomposition rate reflected total decomposer abundances – decomposition rate was significantly reduced under pyrethroid application (Linear Mixed-Effect Model, F = 3.38, d.f. = 1053, P = 0.034). (Figure 9).
North Field. Despite significant differences in millipede activity-densities in response to treatment, decomposition rate was not significantly different across treatments application (Linear Mixed-Effect Model, F = 0.4125, d.f. = 580.9, P = 0.66). (Figure 9). It is likely then, that soil mites and collembola overshadow any effect of millipedes on decomposition in this system.
Also, it should be noted that overall decomposition rate was slower in the North Field, likely due to the flooding. It is possible that the pesticide residual time was reduced by the flooding, reducing their effect.
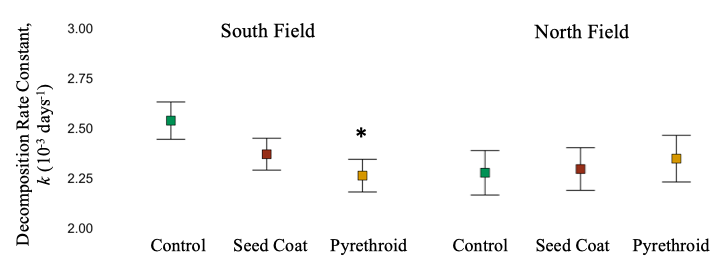
Validity of this Litterbag Study
At the EPFES Workshop, participating scientists proposed a set of criteria for litterbag experiments testing the effect of pesticides on decomposition (Römbke et al., 2003). First, for the experiment to be considered valid, the control litterbags must reach 60% mass loss by the end of the experiment. Second, a treatment is considered concerning/a risk when mass loss is reduced by more than 10% compared to controls. Third, recovery by the end of the experiment/season indicates acceptable risk.
For this experiment, I achieved at least 60% mass loss in the controls for 8/10 batches of litterbags I deployed; the two that did not reach 60% were in the flooded North plot and were close, at 61% and 63% mass loss in the controls. Thus this overall experiment can be considered valid.
However, mass loss under the two pesticide treatments were never reduced by more than 10% from the control. The greatest differences from the control were 5% for the neonicointoid seed coat, and 9% for the pyrethroid. When considering the high variance at these timepoints, the statistical differences are even smaller. Additionally, the treatments converged at the end of each batch of litterbags. So despite statistically significant differences in decomposition rate and significant reductions in decomposer activity, both pesticide treatments are of low (acceptable) risk to decomposition in the field.
Nutrient Mineralization:
South Field. There was no significant difference in litter protein:lignin ratios across treatments (Linear Mixed-Effect Model, F = 0.16, d.f. = 135.97, P = 0.85). Pesticide treatments also had no measurable effect on any measured soil metrics (organic matter content, soil pH, cation exchange capacity, phosphorus, magnesium, potassium, calcium, sulfur, copper, and zinc). As bulk soil nutrients aren’t very sensitive measures of decomposition, it is not surprising we did not observe significant changes in soil nutrients. While it does appear that soil organic matter was accumulating in both pesticide treatments (Figure 10), these measurements were not significantly different than the control. It is likely that two years (May 2017 – Nov 2018) is not enough time to detect significant differences in soil organic matter accumulation.
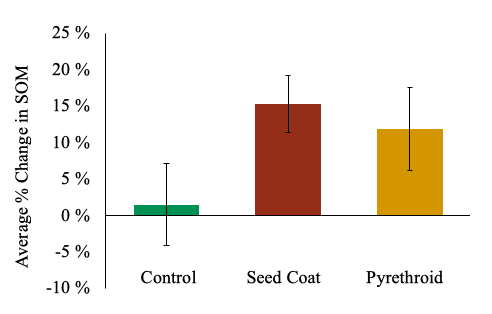
Additional Results
Timing of Litterbag Placement
I placed batches of litterbags in both June and November, to reflect when plant residue would be deposited in these fields – June to reflect cover crop burndown, and November to reflect crop residue after harvest. Despite identical collection timepoints, decomposition rate was consistently higher for bags placed in June compared to November, regardless of field or crop (figure 11). Environmental and biological conditions in early decomposition (e.g. temperature, soil moisture, decomposer community composition/abundance) likely affect the lifetime rate of decomposition.
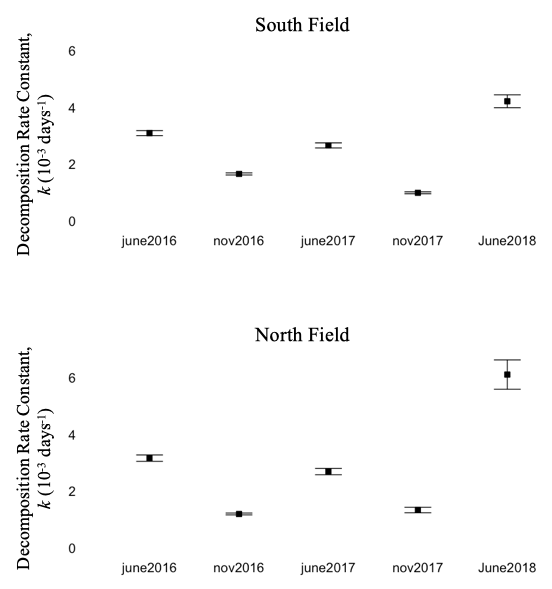
Crop Yield
Across the three years of this experiment, neither pesticide treatment affected corn nor soy yield (Table 2). This would be expected, as insect pest pressures at the field site were low from 2016 - 2018.
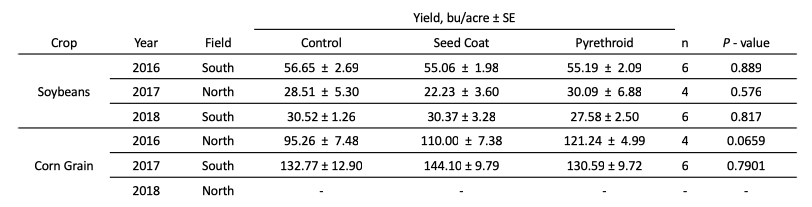
The macro-decomposer community in soybean and corn fields is dominated by a single millipede species, Oxidus gracilis, which appears to have variable population responses to pyrethroid exposure. While specific decomposer populations were reduced by both pesticide treatments, the pyrethroid had greater negative effects on the dominant decomposer group (soil mites). As a result, decomposition was not affected by neonicotinoid seed coats, but was slowed under pyrethroid application. However, while statistically significant, this effect on decomposition was small and therefore unlikely to have a noticeable effect on residue break down.
Soil nutrients and soil organic matter accumulation are not sufficient short-term metrics for determining pesticide effects on decomposition. However, pesticides that subtly slow decomposition may affect soil nutrients and soil organic matter on a longer time scale, which could degrade soil quality.
Despite the negative effects of these pesticides on decomposers and decomposition rate, neither insecticide increased crop yield. At least in the Northeast, pest insect populations usually fall below treatment thresholds, and excessive insecticide application may reduce the effectiveness of sustainable management practices. Overall, these results provide additional justification for growers to reduce unneeded insecticide applications in field crops.
Education & Outreach Activities and Participation Summary
Participation Summary:
I have been able to share my research with farmers and members of the community, and my peers in entomology. I gave a talk about my research to farmers and extension agents at the Penn State Annual Agronomic Weed Tour. I have shared my research with people from the community – from gardeners at the Insect Identification and Soil Health Workshop (Penn State Student Farm) to children at school visits and community events such as Wings in the Park at the Snetsinger Butterfly Garden, Discovery Day at the Snetsinger Butterfly Garden, and the Great Insect Fair at Penn State. For the Great Insect Fair, I collaborated with my colleague to create an interactive display to teach people about soil invertebrates and their contribution to agroecosystems. We will continue to expand this display and to use it at future events. Over the past three years, I have also helped mentor 12 undergraduate students hosted by my lab. Finally, I have presented my research at three Annual Entomology Society of America meetings.
Project Outcomes
The main conclusion that can be drawn from this research is that growers in the Northeast (or at least Pennsylvania) may be able to reduce their insecticide application without significant yield loss; insect pest pressures were low in all three years of this study, and insecticide application did not improve yield. By reducing unnecessary insecticide applications, growers could save money, reduce resource use, and decrease their personal and community exposure to toxic chemicals.
Additionally, the pyrethroid we applied did negatively affect decomposers and decomposition rate; while subtle, these results support the use of neonicotinoids over pyrethroids when insecticide application is warranted. While the magnitude of these results was small, this project was only conducted for three field seasons on a small scale. It is possible that we would observe significant negative effects over decades and acres of insecticide use.
Knowledge/Awareness of Sustainable Agriculture
During this project, I really came to understand how all sustainable management practices are interconnected. Adopting one sustainable practice will affect all other aspects of crop management – pest management, nutrient management, timing of planting, etc. For example, adopting cover crops to reduce weed pressures will also affect what invertebrate pests are present (and how they should be managed). Cover crop choice may also alter natural enemy populations, which may affect how invertebrate pests are managed. Cover crops will alter early season nutrient and water availability, and can shift the best time to plant. It is important for researchers and growers alike to understand how sustainable practices can affect seeming unrelated aspects of crop management.
I also learned why there is no set of sustainable agriculture rules that can be applied in any situation. One of the fields I was working in was ‘normal’ – it was level, drained well, and had moderate weed pressure. The other field was low-lying, prone to flooding, very weedy, and had higher macro-decomposer and pest abundance. Planting time, weed management, expected yield, and decomposition rate were always different for these two fields, despite being less than 1000 feet apart. Had we treated the fields identically, we would have excessively applied herbicide in the ‘normal’ field. Also, pesticides are likely to lose their efficacy in wet fields – a sustainable grower would likely decided to plant something that requires less chemical management to avoid having to apply more pesticides with greater risk of pesticide runoff.
Sustainable agriculture also means being adaptable to changing field management from year to year; 2018 was a very wet year compared to 2016 and 2017; in 2018, we planted later than usual which meant we had to put more effort into weed management. By using cover crops we could reduce weed pressure in all years, but they were especially valuable for the 2018 season.
Research Generated
To follow-up on this work, I applied for a NIFA AFRI Pre-Doctoral Fellowship. The goal of the research I proposed is to tease apart how the fungicides and insecticides in seed coats independently affect decomposers. As noted above, this current project got me thinking about how sustainable management practices are interconnected. Thus I also included a crossed herbicide treatment to determine how herbicides may influence the response of decomposers to pesticide seed coats. As field conditions were variable in this current project, I decided to include a more controlled microcosm experiment in addition to a field experiment.
Future Career and Research Direction
I am aiming for a career as a research scientist in pesticide monitoring, toxicity testing, and regulation. Such a career will likely take me to the US EPA Office of Pesticide Programs, the US EPA regional offices, or state agencies such as the California Department of Pesticide Regulation.
Through this current project, and the research it generated, I will learn the most up-to-date methods to measure functional endpoints and run microcosm studies to understand trophic influence on toxicological outcomes. This research path has allowed me to focus on the theory behind pesticide ecotoxicology, the statistics and interpretation of such studies, and understand how the results from such studies can be applied to pesticide registration, regulation, and grower recommendations.
As detailed in the Results and Discussion section, certain aspects of this project presented challenges.
First, the use of coarse versus fine mesh litterbags to include/exclude macroinvertebrates didn't generate differences in macroinvertebrate access to litter. Size exclusion of invertebrates is challenging and may not have much ecological meaning, as larger invertebrates (e.g. millipedes) will be excluded from fine mesh litterbags as adults, but not as juveniles. Additionally, if the fine mesh bags did excluded macro-decomposers, they would also exclude predators; this could disrupt trophic-interactions and misconstrue conclusions about the role of macro-decomposers. This failure of macroinvertebrate exclusion is largely why I will study macro-decomposers using microcosms in future related experiments.
Additionally, I ran into challenges when running toxicity assays on millipedes and isopods. While methods have been developed for standard test organisms such as earthworms and honeybees, these methods do not translate well for millipedes and isopods. With most of my time dedicated to field work and processing samples, I did not have much time to develop toxicity assays. By the time I had working methods, the field season would be over and the millipedes I had collected were dead (they have an annual life cycle, with adults dying soon after laying eggs in early fall). If I were to do this project again, I would have kept field work to just one field (as initially proposed), which would have given me enough time to trouble-shoot toxicity assays.