Final report for GNE18-170
Project Information
Cover crops (CC) reduce soil erosion, enhance soil fertility, increase water infiltration, and scavenge excess nutrients from soils. It is expected for CC to enhance nitrogen uptake and cycling for subsequent crops in fields that are fertilized with manure. However, there is little information as to how CC nitrogen scavenging is affected by tillage regime and timing of manure application in addition to how CC may impact the rate at which gaseous nitrogen and carbon species are lost from agricultural soils. The objectives of this study were to assess the impacts of the presence or absence of CC, no- tillage or conventional-tillage, and spring or fall manure application on (i) nitrous oxide, ammonia, and carbon dioxide emissions; (ii) CC plus corn (Zea mays L.) nitrogen uptake for the growing season of 2018 in a continuous corn silage system, and (iii) quantify microbial target gene abundance responsible for N2O production two weeks before and after fall manure application. Greenhouse gas measurements were taken via static chamber method twice a week, on average; soil inorganic-N measurements were taken at the time of greenhouse gas sampling. Ammonia measurements were recorded three times within 48 hours of spring and fall manure application, although we experienced measurement errors when compared to related literature by underestimated fluxes. There was little evidence for effects of CC on inorganic-N retention as well as manure-N uptake. Manure-N uptake was generally greatest with spring application. Conventional-tillage reduced ammonia volatilization and retained greater nitrate concentrations relative to no-tillage. We also found evidence for the nitrite reductase enzymes, coded by the nirK and nirS genes, to be 50 to 200 times in greater abundance, respectively, than the nitrous oxide reductase enzyme, coded by the nosZ gene, which reduces N2O to inert N2. However, we did not find strong evidence for soil management practices to greatly alter microbial N2O functional gene abundance. The effects of CC on nitrous oxide and carbon dioxide emissions were large, with the presence of CC enhancing both emissions. Despite this finding, we still recommend CC and no-tillage practices for their vast benefits on soil fertility, water quality, and ability to capture and sequester atmospheric carbon.
Objective 1: Quantify N2O, NH3, and CO2 emissions from a continuous maize system under a combination of CC versus no CC, NT versus CT, and application of spring manure versus fall manure.
Hypothesis 1: Tillage will have the greatest impact on CO2 emissions, with NT plots having the lowest emission by lessening the amount of oxygen introduced to the soil and by protecting labile organic matter in larger soil aggregates. Further, NT plots that include CC are expected to reduce all three emissions by incorporating excess C and inorganic-N into biomass. Relative to fall manure application, manure applied in the spring will decrease CO2 and N2O emissions as crop nutrient demand will be greatest, though it will increase NH3 emissions due to greater solar radiation and atmospheric temperature.
Hypothesis 2: Weather events (e.g., presence or absence of precipitation, above average temperatures, strong winds at the time of manure application, etc.) will be the largest factor affecting all gas emissions and CC productivity, regardless of treatment.
Objective 2: Quantify above and belowground CC biomass as well as CC and corn N uptake from NT and CT plots with application of spring or fall manure.
Hypothesis 1: Cover crop biomass and N-content will be greatest with fall manure application as CC seeds are sewn shortly after that period, thereby providing the crop with adequate nutrients for rapid growth before soils freeze. Tillage will have less of an effect, though CT will promote greater CC biomass by loosening the soil for ease of root growth.
Objective 3: Gain a rigorous understanding of the molecular mechanisms responsible for N2O fluxes before and after manure application with respect to the presence or absence of CC and the presence or absence of tillage via reverse transcription polymerase chain reaction (RT-PCR).
Hypothesis 1: N2O fluxes will be greatest after manure application, regardless of treatment; however, CT plots without CCs will have the largest fluxes relative to NT plots with CCs as more residual nitrate will be available for denitrification and because labile C substrates will be liberated after tillage as a result of breaking apart soil aggregates.
Hypothesis 2: N2O fluxes will be greatest after manure application, regardless of treatment; however, NT plots will favor denitrification (and thus N2O production) relative to CT plots due to the greater soil water content.
Land use conversion for agriculture and traditional cultivation methods causes depletion of the original soil organic carbon (SOC) pool by 60% to 75% (Lal, 2004). Because carbon (C) storage in soils depends on a consistent source of C inputs from plants, loss of SOC with conversion can be largely attributed to a majority of crop biomass being harvested with little plant residue returned to the soil as organic matter. Adequate soil organic matter (SOM) content is essential to soil fertility and proper plant growth for two main reasons: (1) as a majority of SOM is derived from decomposed plant residues, organic matter contains all of the essential nutrients to be recycled for subsequent plant growth; (2) humus (stable organic fraction of SOM) adsorbs bioavailable nutrients for plant uptake and prevents nutrient leaching and loss (Bot and Benites, 2005). Removing plant residues thus increases the need for synthetic or organic fertilizers to replenish soil fertility for successive crops. Besides the negative effects of losing essential bioavailable nutrients, leaving soils bare during the off-season increases the risk of soil erosion and the potential for sediment bound nutrients, primarily soluble phosphorous and nitrogen (N), to leach from manure fertilizers into freshwater systems, or be lost to the atmosphere as nitrous oxide (N2O) or ammonia (NH3) via microbial or abiotic processes, respectively (Duncan et al., 2017; Terman, 1979). In all, because of the degraded soil quality from nutrient and SOC losses, crop biomass yields for subsequent harvests will be reduced, creating a feedback loop requiring increased nutrient inputs (Wightman et al., 2015).
In tandem with SOC losses from conventional land management practices, the agricultural industry is the second largest GHG emitter in the United States (6.3%), next to energy (86.6%) (US EPA, 2007), and is responsible for 30% of global GHG emissions (FAO, 2012). Specifically, agriculture is responsible for approximately one-third of global net carbon dioxide (CO2) emissions primarily due to deforestation and biomass burning, but also significant fluxes being contributed to SOC mineralization and decomposition (Flach et al., 1997; Lal, 2004). Agricultural practices are also responsible for approximately 60% (~ 6 Tg N2O-N) of global anthropogenic nitrous oxide (N2O) emissions, with emissions increasing from about 270 ppb during the pre-industrial era to 319 ppb in 2005 (Snyder et al., 2009). Nitrous oxide is a potent GHG with a global warming potential 265–298 times greater than CO2 for a 100-year timescale (IPCC, 2007), has an atmospheric lifetime of 114 years (IPCC, 2001), and catalyzes stratospheric ozone depletion (Reay et al., 2012); thus, N2O largely contributes to a warming climate. Nitrous oxide emissions are especially prevalent in agricultural soils after application of N fertilizers and cropping practices (Smith et al., 2008; Signor et al., 2013).Indeed, in the United States alone, these same attributes cause approximately 78% of total N2O emissions (US EPA, 2007). Soil N2O emissions are principally a result of two microbial processes – autotrophic nitrification and heterotrophic denitrification (Fowler et al., 2013). Processes that control nitrification and denitrification are directly correlated to SOC, nitrifiable N, temperature, pH, aeration, and soil drainage (Livesley et al., 2008; Mørkved et al., 2007) – all of which are influenced by factors including crop residue, soil porosity, moisture, and aggregate stability (Robertson and Groffman, 2007).
Aside from GHGs, agriculture is the primary source of ammonia (NH3) volatilization to the atmosphere, accounting for more than 50% of the global ammonia volatilization, and N fertilizer applications to crops account for the majority of this atmospheric pollutant (Gordon and Schuepp, 1994; Kaye et al., 2010; Pain and Thompson, 1988). The rate at which ammonia is lost from manure-N is largely dependent on temperature, wind, precipitation, ammoniacal N content of the manure, manure pH, manure moisture content, and the exposed manure surface area (Rotz and Oenema, 2006).
Though agriculture is a major source of GHG emissions and other nutrient losses, changes in agricultural management practices such as cover cropping, no-till (NT), and appropriate timing of manure application are promising solutions for environmental degradation abatement. Cover crops (CC), a BMP used by farmers since the early 1900s, have the potential to benefit agroecosystems in numerous ways. These benefits include reduced soil erosion, increased soil fertility, increased above and belowground biodiversity, pest management, weed control (Lu et al., 2000), and increased residual manure-N uptake (Cambardella et al., 2010). Bare soils are at greatest risk of erosion as a consequence of rainfall impact and high winds. With CC, the velocity of rainfall is impeded by vegetative cover that protects the soil and results in far less soil displacement (Kaspar et al., 2001). Furthermore, CC roots give the soil a stronger foundation, much like scaffolding in a building, thereby reducing soil erosion by holding soil aggregates in place (Romkens et al., 1990). By increasing vegetative biomass, CC reduce the need for herbicide inputs by outcompeting weeds for sunlight, space, and nutrients (Kaye and Quemada, 2017). Soil fertility will be improved from the organic matter supplied after CC senescence (Duxbury et al., 1989), provided it is returned to the soil and not burnt or harvested for sale. Monoculture agriculture has led to increased pest resistance since biodiversity is greatly reduced; introducing a new vegetative species during off seasons allows for other, non-pest, organisms to compete with crop pests for existing ecological niches (Freemark and Kirk, 2001). Competition for resources such as sunlight, space, and nutrients assures that one pest population will likely never evolve to become too dominant. Lastly, of most recent interest would be the use of CC as a tool to improve manure-N efficiency by incorporating excess nutrients from the previous fertilizer application into shoot and root biomass. Cambardella et al. (2010) found that CC reduced NO3-in the surface soil compared to no CC by 32% and 67% after manure application in November 2005 and 2006, respectively.
Adopting NT practice can reduce soil erosion (Oades, 1984), improve soil quality (Zinati et al., 2017), increase water retention (Copec et al., 2015), provide stable or improved crop yields (Bernstein et al. 2011), generally increase soil C gain (West and Post, 2002), and improve overall cropping system function (Snapp et al., 2005). No-till also minimizes soil disturbances which would otherwise increase the risk of erosion and introduce oxygen (O2), an electron acceptor thermodynamically favored over all other microbial respiration pathways, to the system, thereby increasing soil C loss (as CO2) through enhanced microbial decomposition (Alvarez, 2005). Additionally, adopting a NT soil management practice can directly mitigate CO2emissions compared to conventional tillage (CT) by sequestering more C in the uppermost soil layer and indirectly reduce emissions by omitting the need to use fossil fuel-based farming equipment (Alvarez, 2005; Frye, 1984). According to Socolow and Pacala (2007), expanding NT practices could prevent the release of 25 billion tons of C over the next 50 years. Aside from CO2emissions, several studies have shown increases in N2O emissions under CT systems (Koga, 2013; Mutegi et al., 2010; Plaza-Bonilla et al., 2014; Wang and Dalal, 2015), though these results prove to be variable and inconsistent at this time (Lognoul et al., 2017).
No-till systems also have positive impacts on biotic soil communities, which are critical in managing nutrient cycling and thus soil fertility (Wu et al., 2015).Under a CT system, soil biodiversity is indirectly negatively altered by changes in physical and chemical properties such as SOM, soil moisture, soil temperature, and aeration – all of which control soil microbial activity (Sheibani and Ahangar, 2013).Through the decomposition of SOM, microorganisms (less than 0.1 mm in diameter) are responsible for the gradual and continuous liberation of plant nutrients via mineralization and immobilization of N, P, and sulfur (Duxbury et al., 1989). In organic soils, available nutrients that are not taken up by the plants are immobilized by soil organisms and consequently mitigate leaching (Rice, 2002).
Lastly, it is known that climate is a major influence on the rate of GHG emissions by regulating soil temperature, soil moisture, and crop growth rate (Goh, 2004; Powlson et al., 2014). For these reasons, the time of year in which manure is applied can substantially impact the rate of uptake or transformation of C and N substrates (Linn and Doran, 1984). However, studies that have observed fall manure application compared to spring have typically only quantified N2O losses and show no general consensus. Duncan et al. (2017) found that applying manure in the fall as opposed to spring can significantly decrease N2O fluxes yet still provide adequate nutrients to the following CC. On the contrary, Rochette et al. (2004) found greater potential for increased N2O emissions with fall manure application. They attribute these findings to (i) addition of N when crop uptake is low; (ii) low evaporation in the fall typically results in greater soil moisture, favoring denitrification; (iii) the potential for denitrification to continue during the winter; and (iv) fall application will supply soils with N and organic C substrates which will promote denitrification after snow melt, which is consistent with the findings of Lemke et al. (1998) and Wagner-Riddle and Thurtell (1998).
While agricultural BMP have their benefits, there is little information in the literature for the ideal tillage method in regard to the impacts of CC on manure-N uptake. Indeed, there may not be a BMP without tradeoffs among GHG fluxes, N volatilization, C sequestration, and soil fertility. For example, Lognoul et al. (2017) concluded that higher SOC and total N in the uppermost soil layer in a NT field of seven years contributed to increased N2O and CO2emissions compared to the CT treatment. For N2O emissions, several studies reported higher fluxes from NT compared to CT (Abdalla et al., 2013; Ball et al., 2008; D’Haene et al., 2008; Goossens et al., 2001), where other studies have shown no significant correlations between the tillage treatments (Chatskikh et al., 2008; Negassa et al., 2015). A large majority of these studies contribute the escalated emissions chiefly to increases in labile C substrates for decomposition. Of further conflict, in a review of manure application methods, Webb et al. (2010) found that incorporating manure into the soil profile directly after application decreased NH3 losses by as much as 78 to 99% in comparison to broadcast application. These findings can pose large concerns for nutrient losses as NH3in NT systems where manure will be broadcasted onto the soil surface without incorporation into the soil profile. In general, the net effects of GHG emissions and volatile N losses from NT and CT systems with different manure management methods are inconsistent and not well quantified at a global scale (Cassman et al., 2003).
It is clear that there is an urgent need to develop intergraded and sustainable approaches that will improve the nutrient use efficiency of crops in order to alleviate gaseous nutrient losses from agricultural soils without compromising soil fertility. Within this framework, it is fundamental to develop the ideal tillage, off-season cropping, and manure management solutions for farms that grow corn silage in northern regions as currently more than half of the land for corn silage production is located in a zone with low temperatures from -28 to -33 °C. Furthermore, the relationship between CCs, the amount of biomass they can achieve, and the amount of manure-N that they can accumulate for the following cash crop is not understood under northern climates at this time. Similarly, there is little information amongst the literature on the interactions of tillage, manure application timing, and CC systems on GHG emissions and volatile N losses. In all, farmers that are able to successfully combine the use of NT and CC into a robust NT production system are expected to enjoy improved revenue and environmental quality for their farm and the public good.
Cooperators
Research
Site Description
The field trial was established at Borderview Farms in Alburgh, VT (lat. 45.005, long. - 73.308) in fall 2017 as a continuous corn silage system. Prior to 2017, the field was conventionally managed with spring manure application with incorporation via moldboard plow and a winter CC of winter rye (Secale cereale). Soils at this site are classified as a Benson rocky silt loam (Soil Survey Staff, 2017). The experimental design is a randomized complete block design with four blocks. Plot sizes are 4.3 m wide by 24.4 m long, with a 12.2 m buffer between them. Each block contained all combinations of three treatments: tillage (conventional versus no-tillage), manure application timing (spring versus fall), and CC (presence versus absence). There was a total of 8 plots per block for a total of 32 plots. For the conventionally-tilled plots, manure was broadcasted and incorporated within an hour of application via moldboard plow. For the no-tillage plots, manure was broadcasted and remained on the soil surface. Liquid dairy manure was applied in the fall or the spring at 7,000 gallons per acre. For the tilled plots, manure was applied and incorporated the day of application. For the no-till plots, manure remained on the soil surface. Within two days, winter rye CC was drilled at a rate of 110 pounds of seed acre-1. Cover crops were terminated with a moldboard plow in the conventional-tillage plots or with glyphosate in the no-tillage plots.
Gas Measurements
To compare the impact of management practices on GHG (N2O and CO2) emissions, we measured CO2 and N2O emissions from soils within all 32 plots using the static chamber method following the USDA–ARS GRACENET protocol (Parkin and Venterea, 2010) with a vented polyvinyl chloride (PVC) lid (30 cm inner diameter and 9.5 cm inner height) on a chamber collar (30 cm inner diameter, 15 cm height) with an air-tight elastic seal over the static flux chamber collars. Collars were installed to a depth of 12 cm such that the height of the collar above the soil surface was ~ 3 cm (Parkin and Venterea 2010). Vegetation growing inside the chamber collars was cut to ground level before GHG measurements to avoid erroneous CO2 measurements from plant respiration. GHG sampling occurred every other week, on average, with more frequent sampling after manure application and precipitation events; sampling occurred from April 22, 2018 to October 31, 2018 (i.e., when soils were not frozen). GHG samples (10 mL) were collected with polypropylene syringes from the PVC lid through a small butyl stopper at time 0, 15, 30, and 45 minutes after chamber lid deployment; samples were immediately stored for analysis in 6 mL pre-evacuated vials fit with butyl rubber stoppers. GHG concentrations were analyzed using a GC-2014 Gas Chromatograph (Shimadzu Instruments, Kyoto, Japan) equipped with a flame ionization detector (FID), electron capture detector (ECD), and a Hayesep N 80/100 Mesh 1/8in X 1.5M stainless pre-conditioned column. Samples were introduced into the gas chromatograph using an autosampler. Flux rates were calculated from a linear regression of the respective GHG concentration versus the time since chamber lid deployment
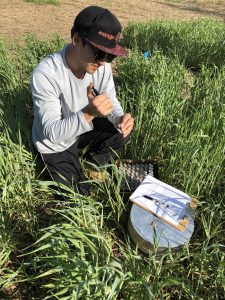
Ammonia measurements were also analyzed using static flux chambers and an infrared photoacoustic spectroscopy (PAS) gas analyzer (Model 1412i, Innova Air Tech Instruments, Ballerup, Denmark; calibrated as in Iqbal et al. 2013). Ammonia fluxes were measured at 0, 24, and 48 hours after each manure application, as NH3 volatilization is the greatest within 48 hours of liquid manure application (Dell et al., 2012; Duncan et al., 2017, Liu et al., 2018; Thompson and Meisinger, 2005). The NH3 concentration was recorded every minute for four minutes by placing a vented PVC lid on the chamber collar (as above) connected in a closed-loop system with the PAS gas analyzer, which measures gas concentrations non-destructively. Linear regressions was used to calculate flux rates (i.e., slopes); slopes that were not significant (p < 0.1) were set to zero.
Soil Sampling and Analysis
Soil samples (2 cm inner diameter, 0-15 cm) were collected no further than a meter behind the chamber collar during each GHG sampling event. Samples were placed in polyethylene bags, homogenized, and kept on ice for transport to the lab for analysis. Within 24 hours of sampling, a 5 g soil subsample was extracted with 2 M KCl for inorganic-N (NO3- and NH4+) quantification. The resulting KCl solution was frozen for coulometric analysis with a BioTek Synergy HTX (BioTek Instruments, Inc., Winooski, Vermont, USA). A second 5 g subsample was extracted with deionized water to determine nitrite (NO2-) concentrations, a substrate with the potential to produce N2O via nitrification, following the methods as described in Giguere et al. (2017); NO2- was analyzed colorimetrically directly after extraction on a BioTek Synergy HTX. Soil NO2- was continuously below detection for three sampling events and therefore was not further analyzed or further discussed. A third 5 g subsample was dried at 60 °C to a constant weight to determine gravimetric soil moisture. Additionally, we recorded soil temperature at the time of GHG sampling. Measurements were taken adjacent to chamber collars so as to not disturb soil within the chamber.
Cover Crop Analysis
Prior to spring manure application and CC termination, plots were assessed for CC cover using the Canopeo application, in accordance with the protocol of Patrignani and Ochsner (2015). The CC height was measured at three random locations in each plot measuring to the highest point in cm. The material in two quadrats (0.25 m2) in each plot were cut to ground height, placed in a cloth bag, weighed, and dried at 60 °C. After drying the material, samples were weighed again to determine dry matter content and dry matter yield (g m-2). The samples were then ground to 1 mm in order to analyze for TC and TN content by combustion (LECO CHN628). The same process was repeated directly before planting corn. Aboveground corn biomass was also analyzed for TN content (as above).
The soil core method was used to assess belowground CC biomass to a depth of 30 cm. Three samplings points were randomly selected in plots where CC were present and a soil core (4 cm inner diameter) was used to collect CC root samples. A metallic sieve was used to wash all soil from roots with deionized water, which was then air-dried to remove added moisture, weighed, and dried at 60 °C until a constant weight was achieved. Dried samples were reweighed and ground. Ground CC roots were analyzed for TC and TN content by combustion (as above). The average of the three root biomass samples was extrapolated to the area of the plot using the dry weight of the root samples collected to the depth of the core (g m-2). Total CC biomass was determined by summing the above and belowground yields. Corn and CC N uptake were quantified by the TN content and yield of each crop per unit area (g N m-2). Corn and CC N uptake values were summed for one representative value, if CC were present.
N2O Functional Gene Abundance Analysis
The fate of soil N is almost entirely dependent on reduction-oxidation reactions primarily controlled by soil microorganisms. It is well known that N2O is a byproduct of nitrification and denitrification, which is mediated by autotrophic nitrifying and heterotrophic denitrifying bacteria, respectively. The first step of nitrification requires ammonia-oxidizing bacteria to oxidize ammonia to nitrate. Nitrification is typically studied using the marker gene amoA, coding for the alpha-subunit of the AMO enzyme, because its nucleotide sequence is highly conserved amongst this functional group and due to its potential to clarify the effect of the amoA gene community structure on N2O flux rates. Denitrification is a multi-step process that reduces nitrate to inert N2 gas with a key enzyme being nitrite reductase (Nir), which catalyzes the reduction of soluble nitrite into NO (the precursor to N2O) (Németh et al., 2014). This reaction can be carried out by two Nir enzymes: a copper nitrite reductase encoded by the gene nirK or cytochrome cd1-nitrite reductase encoded by the nirS gene. Lastly, if denitrification is complete, nitrous oxide reductase, coded by the nosZ gene, will completely reduce N2O to N2 (Henry et al., 2004).
Nitrous oxide target gene abundance analysis occurred on soils sampled two weeks before and after manure application. Soil subsamples (0.25 g) were stored at -80 °C until RNA extraction. RNA was extracted with Qiagen RNeasy PowerSoil Total RNA Kit where a quality check was immediately performed on a NanoDrop spectrophotometer (NanoDrop Technologies, Wilmington, DE) after extraction. Standards and primers were selected based on the gene sequences of interest (i.e., amoA, nirK, nirS, and nosZ). Extracted RNA samples and standards were ran in triplicates on a Roche 480 LightCycler. The reverse transcription polymerase chain reaction (RT-PCR) was performed in a Mastercycler ep realplex real-time PCR system (Eppendorf North America, Inc., Hauppauge, NY) using the following conditions: initial heat denaturation at 95° C for 2 min, followed by 30 cycles consisting of a denaturation step at 95° C for 5 s, annealing at 56° C for 15 s and extension at 72 °C for 20 s. The amounts of amoA, nirK, nirS, and nosZ gene copies in the soil were calculated on an oven- dry weight (60 °C) basis.
Statistical Analysis
Daily N2O, CO2, and NH3 flux rates, CC biomass and crop N uptake, and N2O functional gene abundance were analyzed using linear mixed models that included (1) plot as a random effect to account for non-independent measurements from the same plot over time, (2) date as a factor to examine how treatment effects changed among days, (3) a constant variance function to account for heterogeneous errors among the tillage, manure application season, and/or CC treatments (as needed to meet homogeneity of error assumptions), and (4) two, three, and four way interactions among tillage, manure application season, CC, and date. Flux, CC biomass, and gene abundance data were transformed as needed to meet normality assumptions: N2O and NH3 fluxes were cube root transformed; CO2 fluxes were square root transformed; CC biomass data were log transformed.
To examine the impact of hypothesized drivers on daily GHG fluxes, including soil inorganic-N (NO3- and NH4+), soil temperature, and soil moisture, we added them as covariates without interactions (α = 0.05) to the above models. Akaike’s Information Criterion modified for small sample sizes (AICc) was used to select the best covariates for explaining each variable. To choose the best model(s) we considered models with dAICc ≤ 2 to have substantial support, where dAICc is the difference between the model under consideration and the model with the lowest AICc value (Burnham and Anderson, 2002). When no single model was best, we chose the simplest model with a dAICc < 2 (i.e., the model with the fewest independent variables).
All linear mixed effects models were fit using the nlme package in R Studio (R Core Team 2018; RStudio Team 2015; Pinheiro et al., 2018). We calculated marginal and conditional R2 values using the piecewiseSEM package in R (Lefcheck, 2015). Marginal R2 describes the proportion of variance explained by fixed factors alone (i.e., manure application season, tillage, CC, date, and interactions), while conditional R2 describes the proportion of variance explained by fixed and random factors (fixed factors plus plot; Nakagawa and Schielzeth, 2013). Treatment significance was assessed using F tests.
Results
Daily N2O, CO2, and NH3 emissions
Daily N2O emissions were significantly affected by CC, but the impact of this treatment varied by date (significant two-way interaction; p < 0.05). The season of manure application also significantly impacted N2O fluxes, but its impact varied with date and tillage treatment. The presence of CC increased daily N2O emissions, though only after manure application and heavy rainfall events (Fig. 1). Overall, the presence of CC increased average N2O emissions by 2.7 times relative to CC absence throughout the study duration. With respect to manure application season, fall manure application increased average N2O emissions by 1.5 times relative to spring manure application (Fig. 1). In general, N2O emissions from tillage treatments alone were small and varied by date. Our measured covariates (i.e., soil moisture, soil temperature, and inorganic-N) did not have a significant impact on daily N2O emissions.
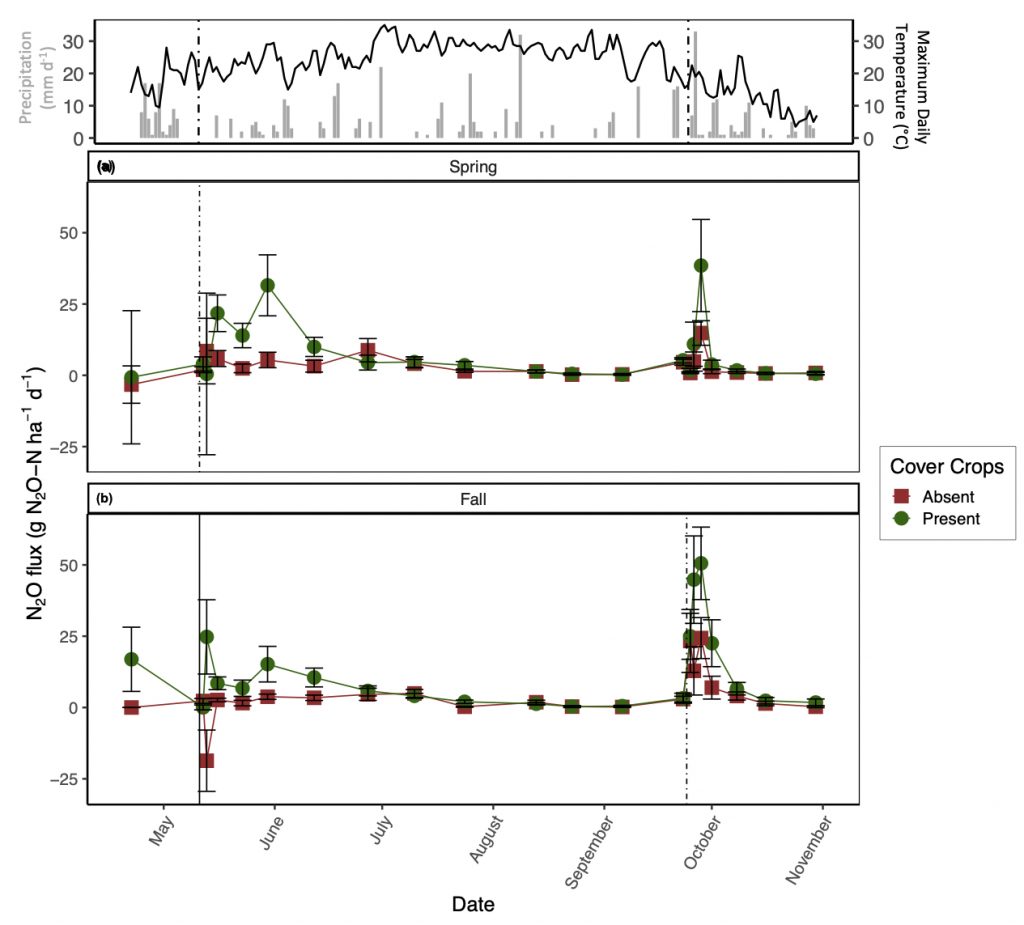
The presence of CC increased daily CO2 emissions relative to CC absence, but this difference decreased throughout the growing season (significant CC by date interaction; Fig. 2). On average, the presence of CC increased CO2 emissions by 1.5 times relative to the absence of CC (Fig. 2), although CO2 fluxes were variable throughout the sampling duration and largely coincided with atmospheric temperature (Fig. 2). The greatest pulse of CO2 from the spring manure application plots was recorded directly after tillage and manure application with the presence of CC (43 ± 8.8 kg CO2-C ha-1 d-1); whereas, the greatest pulse for fall manure application was recorded before CC termination (38.3 ± 4.9 kg CO2-C ha-1 d-1; Fig. 2). Although manure application season by date was a significant predictor variable for CO2 production (p < 0.05), average CO2 emissions only increased by 1.1 times with spring manure application relative to fall manure application (Fig. 2). From the daily CO2 ANCOVA, soil temperature and NH4+ were significant predictor variables for daily CO2 production (marginal R2 = 44%). In general, CO2 flux increased with soil temperature and soil NH4+ regardless of treatment.
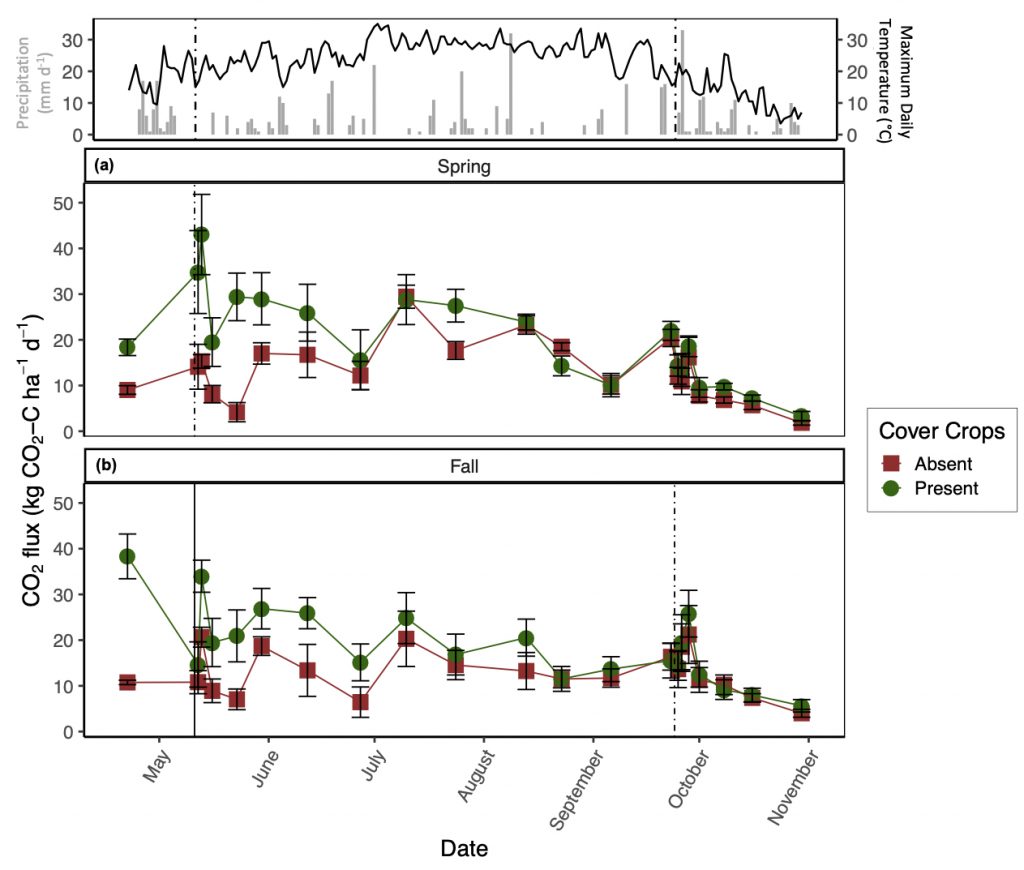
Post manure application NH3-N fluxes were greatest immediately after manure application and in no-tillage but were higher in the spring versus fall manure application treatment (significant tillage by season by date interaction). On average, no-tillage increased NH3-N emissions by 3.2 to 31.8 times relative to conventional-tillage, with conventional-tillage typically acting as a sink for NH3 (Fig. 3). On average, spring manure application increased NH3-N emissions by 1.9 to 10.5 times relative to fall manure application (Fig. 3). None of the measured covariates had a significant impact on NH3-N emissions. Total NH3-N emissions from our study were substantially smaller than emissions reported in studies with similar manure application rates and manure NH4-N content, such as in Dell et al. (2012) and Duncan et al. (2017). We found that the greatest NH3-N (813.9 ± 346.4 g NH3-N ha-1 d-1) loss occurred at time 0 with spring manure application, yet only accounted for a loss of 1.6% of manure NH4-N applied. Because of these measurement concerns, NH3 data were used only to compare differences amongst treatments on emission rates.
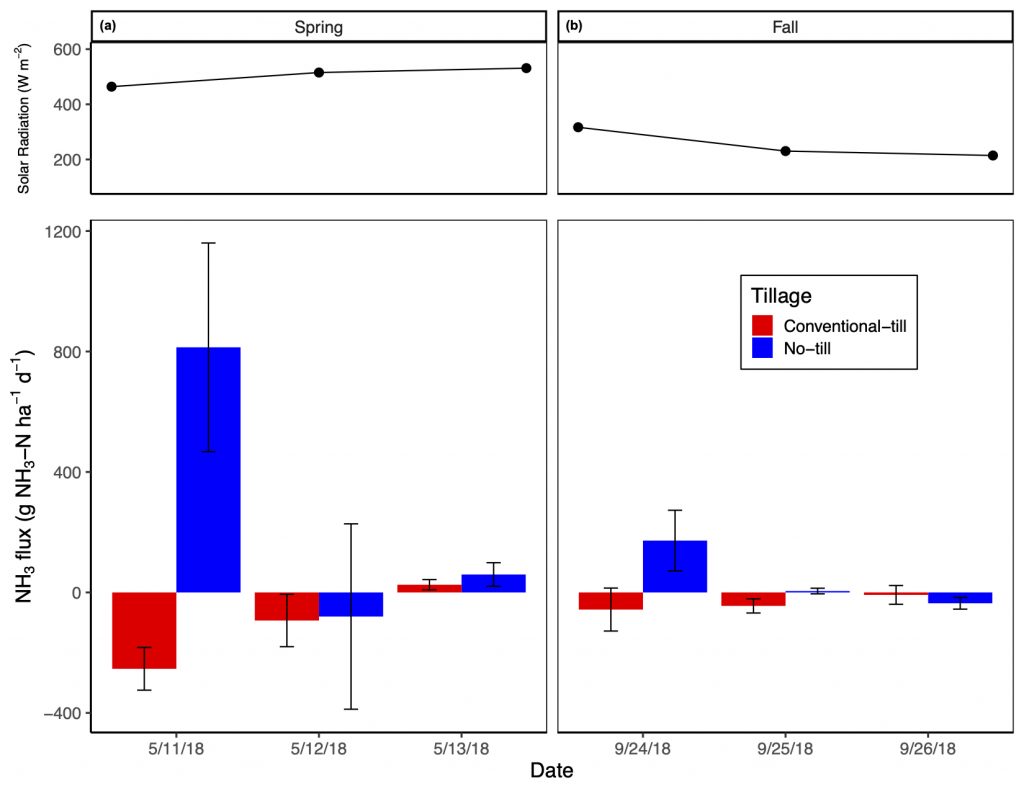
Cover crop biomass and crop N uptake
Above and below-ground CC biomass was not significantly affected by any of our treatments or covariates. In general, total CC biomass was highly variable amongst treatments and ranged from 335 ± 28 g m-2 with no-tillage and fall manure application to 470 ± 115 g m-2 with conventional-tillage and fall manure (Fig. 4a). For crop N uptake (i.e., corn plus CC), tillage regime and manure application season were marginally significant predictor variables (p = 0.07; marginal R2 = 44%). Crop N uptake was lowest in the no-tillage treatment with fall manure application (160.6 ± 11.6 g N m-2) and greatest in the conventional-tillage treatment with spring manure application (196.3 ± 13.1 g N m-2; Fig. 4b). Crop N uptake was also significantly affected by soil temperature (p < 0.05), where an increase in temperature from 16 °C to 19 °C generally decreased crop N uptake.
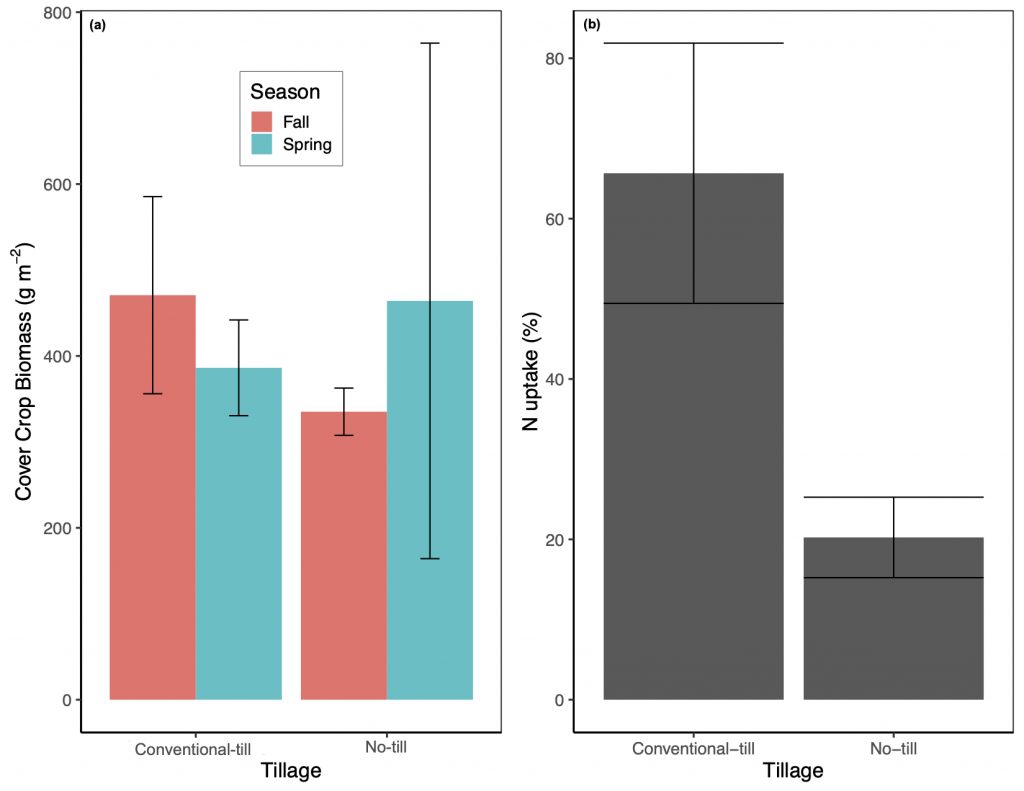
N2O functional genes
The amoA gene, regulating potential N2O losses via nitrification, was significantly affected by a four-way interaction including cover crops by tillage regime by season by date (p = 0.04; marginal R2 = 34%). However, due to the low gene copy numbers (ranging from 7 to 239 starting copies) that are lower than gene copy numbers reported in other studies, we believe that we have underestimated the amoA gene copy abundance. This is likely due to improper primer selection, and therefore the amoA gene copy abundance has been excluded from this study. Therefore, we only aim to identify the potential for target genes relating to denitrification (nirK and nirS) to produce N2O emissions as well as the potential N2O emissions reduction via the nosZ gene by converting N2O back to N2.
The nirK gene, coding for the copper nitrite reductase enzyme, was significantly affected by a four-way interaction including cover crops by tillage regime by manure application season by date (p = 0.02; marginal R2 = 28%). Averaged nirK gene abundance ranged from 86258 ± 30692 gene copies in the conventional-tillage with fall manure application and the presence of cover crops to 208550 ± 99794 gene copies in the conventional-tillage with spring manure plots and the absence of cover crops. In general, nirK gene abundance tended to be greater before fall manure was applied (i.e., on September 14th relative to September 24th; Fig. 5a), with the exception of the conventional-tillage with spring manure application and presence of cover crops treatment. The difference between cover crop treatments was also greatest before fall manure application, where differences amongst treatments became more similar after fall manure application (i.e., September 27th sampling period). After fall manure application, the no-tillage plot with the presence of cover crops had a higher abundance of the nirK gene relative to the same tillage regime with the absence of cover crops; whereas the opposite trend tended to be true with conventional-tillage where the nirK gene copy number was lower with this tillage regime and the presence of cover crops relative to conventional-tillage and the absence of cover crops (Fig. 5a).
The second gene coding for the cytochrome cd1-nitrite reductase enzyme, nirS, was significantly impacted by date (p = 0.02; marginal R2 = 27%); a four-way interaction including cover crop treatment, manure application season, tillage regime, and date had a marginally significant impact on nirS gene copy abundance (p = 0.09). Averaged nirS gene abundance from all treatments ranged from 61431 ± 50339 gene copies from the conventional-tillage with fall manure application and presence of cover crop treatment to 2314764 ± 2286341 gene copies from the no-tillage with fall manure application and absence of cover crops treatment. The nirS gene copy number for all treatment combinations was greater than the nirK gene copy numbers (both genes coding for similar functional enzymes), although variability amongst experimental replications was larger with the nirK gene relative to nirS gene. Gene copy abundance was greater on September 14th relative to September 27th (i.e., before fall manure application; Fig. 5b). Although not a significant finding, the no-tillage plots with the presence of cover crops generally had a greater abundance of nirS gene copies, with the exception of no-tillage and spring manure application on September 27th (Fig. 5b).
To complete denitrification, nitrous oxide reductase, coded by the nosZ gene, will completely reduce N2O to N2. The nosZ gene abundance was significantly affected by a three-way interaction including cover crops by manure application season by date (p = 0.03; marginal R2 = 24%), with a marginally significant four-way interaction including cover crops by tillage regime by manure application season by date (p = 0.07). In general, five out of the eight treatment combinations had greater nosZ gene expression under the absence of cover crops relative to the presence of cover crops (Fig. 5c). The greatest nosZ gene copies (5474 ± 963 copies) were found in the no-tillage with spring manure application and the absence of cover crops on September 27th whereas the lowest gene copies (1400 ± 508 copies) were found in the conventional-tillage treatment with spring manure application and the presence of cover crops on September 14th (Fig. 5c).
Fig. 5: Target gene abundance for (a) nirK, (b) nirS, and (c) nosZ g dry soil-1
Discussion
Impacts of tillage regime, cover crops, and manure application season on GHG emissions
In contrast to our expectations, the presence of CC increased GHG emissions, particularly for N2O and CO2. Indeed, a meta-analysis of the impacts of CC on N2O emissions found that CC often increased N2O emissions relative to fallow treatments (Basche et al., 2014). This finding was primarily attributed to differences in N fertilization rates amongst studies, along with CC termination method, CC type (i.e., legume versus non-legume), and variability in precipitation across regions. Furthermore, Mitchell et al. (2013) found that high C inputs from non-legume CC can stimulate N2O production. Incorporation of CC residues via tillage increases N mineralization rates of both SOM and CC reside, resulting in a release of NO3- to the soil matrix (Firestone and Davidson, 1989). Our results support this finding: we observed the greatest average NO3- concentrations with CC present in the conventional-tillage plots with spring manure application. Parallel to increased NO3- as a result of CC incorporation, incorporation of CC via tillage can increase soil temperature compared to soils with CC reside remaining on the soil surface (Omonode et al., 2011) and promote anaerobic conditions by stimulating microbial decomposition, which depletes soil O2 (Kasper and Singer, 2011). Our results showed that differences in soil temperature between tillage treatments were greatest directly after CC incorporation with this effect lasting until late July (data not shown). Increased soil NO3-, increased soil temperature, and low O2 conditions as a result of incorporating CC residue likely favored denitrification and promoted greater N2O emissions relative to residue remaining on the soil surface or the absence of CC residue. In addition to N2O emissions, when grass CC are incorporated into the soil via tillage, transient increases in CO2 fluxes can be expected as O2 is rapidly mixed in the soil profile along with labile C substrates (Franzluebbers et al., 1995; Reicosky and Archer, 2007) and physical constraints on CO2 diffusion are removed (Abdalla et al., 2014). Such mechanisms may be driving our observation that the presence of CC in a conventional-tillage system promoted the greatest average N2O and CO2 losses irrespective to the season in which manure was applied, with the exception of no-tillage and the presence of CC promoting marginally greater N2O emissions with spring manure application.
To further demonstrate the strong control of CC on N2O and CO2 emissions, the conventional-tillage treatment with the absence of CC showed the greatest reduction in both emissions, suggesting that the addition of C substrate from CC superseded the impact of soil disturbance on N2O and CO2 emissions. Furthermore, the no-tillage treatment with the presence of CC promoted the second greatest losses of N2O and CO2, irrespective of manure application season. We found that soil moisture was generally greatest from the no-tillage plots compared to the conventional-tillage plots, with the greatest difference directly after CC termination (data not shown). Soil moisture affects C mineralization (Chimner and Cooper, 2003; Daulat and Clymo, 1998) and O2 availability in soils (Li et al., 2001), both of which can affect CO2 emissions (Jabro et al., 2008). This finding may explain the lack of significance between the tillage regimes and daily CO2 emissions; although mechanical soil disturbance alone is expected to increase CO2 emissions, the greater C inputs from CC residue incorporation via tillage and the increase in soil moisture from CC residue remaining on the soil surface with no-tillage likely acted as separate mechanisms that both enhanced CO2 emissions. In other words, when CC are present, the production of CO2 from the conventional-tillage plots is likely due to increases in C directly entering the soil, whereas the release of CO2 from the no-tillage plots is likely a result of enhanced soil moisture, both of which can promote heterotrophic decomposition. Indeed, Fontaine et al. (2004) demonstrated that the supply of labile C may accelerate the decomposition of soil C and induce a negative C balance as a result of nutrient competition amongst microbes. Furthermore, when observing the impacts of CC on daily CO2 emissions alone, we observed the greatest difference amongst treatments after CC termination with sustained elevated emissions from the presence of CC lasting until July (Fig. 2).
It is also interesting to note that the greatest daily CO2 emissions with fall manure application were recorded from the CC treatments before termination (Fig. 2b). It is likely that these emissions were mediated by CC root respiration paired with heterotrophic respiration, further demonstrating the impact of CC on CO2 emissions. The lack of significant differences between tillage regime on daily CO2 emissions can alternatively be explained by the site history where the field was previously managed by conventional-tillage, suggesting that there may be a transitionary period in which newly converted no-tillage practices do not show a reduction in SOC losses as CO2. Indeed, Six et al. (2004) compiled all available data from soil-derived GHG emissions comparing conventional-tillage and no-tillage and found that newly converted no-tillage systems increased global warming potential relative to conventional-tillage systems within the first ten years of conversion, regardless of climate regimes, by increasing both N2O and CO2 emissions.
Even though the presence of CC significantly increased CO2 emissions irrespective of tillage regime, it is important to consider the amount of C being captured in CC biomass. Based on the TC content of CC biomass per unit of area, we found that the presence of CC offset cumulative CO2 emissions by 31%, irrespective of tillage regime or manure application season. By tillage regime, CC offset cumulative CO2 emissions by 30% and 33% with conventional-tillage and no-tillage, respectively. In general, CC C uptake was greater with fall manure application relative to spring manure application, likely due to pairing manure amendment at the time of CC seeding. Moreover, West and Marland (2002) described that no-tillage practices can lead to ancillary CO2 emission reductions by as much as 31 kg C ha-1 yr-1 over 20 years by reducing fuel inputs involved with conventional-tillage.
Impacts of tillage regime and manure application season on NH3 emissions
Like GHG emissions, NH3 emissions were measured for comparison amongst treatments and to compare results found in Dell et al. (2012) and Duncan et al. (2017) where two separate NH3 sampling methods were used. The former study used recirculating chambers with passive diffusive acid traps whereas the latter study used a PAS, such as in this study. Duncan et al. (2017) reported that when comparing their PAS data against cumulative loss values measured in Dell et al. (2012), the PAS consistently underestimated total NH3 losses. We also found this to be true with respect to the results produced by both Dell et al. (2012) and Duncan et al. (2017). Duncan et al. (2017) reported NH3-N losses as high as ~18 kg NH3-N ha-1 d-1 three hours post broadcast manure application (i.e., no incorporation); Dell et al. (2012) reported the greatest loss of ~8.2 kg NH3-N ha-1 h-1 directly after broadcast manure application without incorporation, representing a 70% loss of manure NH4-N through volatilization when manure was not incorporated into the soil profile. Both author’s results are consistent to related literature where total NH3-N emissions after broadcast application of manure without incorporation typically accounted for 40 to 60% of manure NH4-N content applied (Bittman et al., 2005; Hansen et al., 2003: Smith et al., 2000), but losses up to 90% have been reported (Wulf et al., 2002). Again, as our observed NH3-N emissions only accounted for 1.6% of manure NH4-N applied, which is likely a large underestimation, we only aim to compare differences amongst treatments. We expect that our NH3-N emissions were markedly lower compared to similar studies as (i) many of our individual flux rates were not significant when assed via linear regression and were therefore assumed to be 0 g NH3-N ha-1 d-1, and (ii) utilizing the PAS to observe NH3 fluxes poses time limitations when attempting to capture measurements from multiple treatments, which introduces large variability in time-sensitive measurements amongst replicates.
Our results are consistent with other studies in that NH3 volatilization is greatest when manure is broadcasted onto the soil surface without any form of incorporation (Al-Kanani and MacKenzie,1992; Mkhabela et al., 2008). Our results also demonstrate that timing of manure application can greatly affect the rate at which NH3-N is volatilized, with spring manure application promoting the greatest losses relative to fall application. Greater losses of NH3-N from no-tillage and spring manure application are likely a result of similar mechanisms. Atmospheric temperature and wind speed were relatively similar between spring and fall manure application, though spring manure was applied during a period of low precipitation, comparatively higher solar radiation (Fig. 3), and had a higher dry matter content compared to fall manure. The combination of low precipitation at the time of spring manure application paired with the greater dry matter content likely resulted in less infiltration of manure NH4-N into no-tillage soils as a result of lower fluidity (Sommer and Olesen, 1991). Indeed, Chambers et al. (1999) found that NH3-N losses from manure NH4-N increased by about 5% for each 1% increase in manure dry matter content. For this reason, it is probable that precipitation and soil moisture facilitate the infiltration of manure NH4-N into the soil profile where it is protected from volatilization by adsorption onto soil colloids. Aside from manure characteristics and precipitation, as spring manure NH4-N remained on the soil surface in the no-tillage plots, it was exposed to greater solar radiation relative to fall manure application (Fig. 3), which promotes greater NH3 volatilization rates. These results suggest that the greatest NH3-N volatilization abetment should be achieved when manure is incorporated into the soil directly after application or, for no-tillage practices with liquid manure broadcast application, applied directly before precipitation events, or high soil moisture, along with low solar radiation. While it is often difficult to pair cropping management events with weather events, advances in manure application methods, such as shallow disk injection and surface banding with soil aeration, now allow farmers to inject liquid manure directly into no-tillage soils where manure-NH4 is not exposed to adverse weather conditions. To demonstrate the efficiency of NH3 volatilization mitigation with subsurface manure application in no- tillage systems, Dell et al. (2012) found that injection decreased NH3-N emissions by 91 to 99% compared to broadcast manure application.
To our knowledge, our study is the first to demonstrate the ability of fallow, conventionally-tilled, soils to act as an NH3 sink, especially directly after manure application and incorporation (Fig. 3). Ammonia has a relatively short atmospheric lifetime and can be rapidly deposited less than 10-100 km from where it was emitted (Asman et al. 1998). In addition, NH3 exists in an equilibrium with NH4+ where the relative concentration of each depends on the pH of the solution it is contained in (Schlesinger and Bernhardt, 2013); equilibrium between the two N species exists at a pH of 9.3, where more alkaline conditions will favor the formation of NH3 (Gupta et al., 2015). The pH of all conventional-tillage plots, regardless of treatment, averaged 7.2, favoring the formation of NH4+ over NH3. As we did not find manure application to greatly increase soil NH4-N concentrations, we expect that the NH3 volatilized from the no-tillage plots may have been captured in the chamber lid while measuring emissions from the conventional-tillage plots and readily deposited on the soil where it was abiotically converted to NH4+. As NH4+ became available, it is probable that nitrifying bacteria became stimulated with the addition of nutrients from manure and O2 from tillage and converted NH4+ to NO3-. This mechanism can be supported by our daily soil NO3- measurements as we observed a more rapid increase in NO3- concentrations with conventional-tillage and spring manure application relative to fall manure application.
Impacts of tillage regime on cover crop biomass and N uptake.
Cover crop biomass was not significantly affected by any treatment or measured covariates. This is likely due to all plots receiving the same manure application rate as Parkin et al. (2006) have demonstrated that rye CC shoot, root, and total dry weight only increased with increasing manure application rates and was not affected by tillage regime (i.e., no-tillage versus conventional-tillage). In contrast to our expected results, the presence of CC did not increase crop N uptake. Differences in crop N uptake were driven by manure application season and tillage regime, though these results were only marginally significant, with spring manure application generally resulting in the greatest amount of N uptake (Fig. 4b). However, N uptake from CC only contributed to 3% to 13.6% of total N uptake, with corn N uptake accounting for the rest. Thus, the greater N uptake with spring manure is likely a result of applying manure directly before planting the cash crop. Parkin et al. (2006) have also demonstrated that rye shoot and root N concentrations only increased with an increase in N application rate and did not significantly differ amongst tillage treatment. Alternatively, as we found the presence of CC to enhance N2O emissions, it is possible that a large portion of manure N was lost via denitrification, even though previous studies have shown that living plants effectively compete with microbes for available NO3- and thus reduce denitrification rates (Haider et al., 1985; Smith and Tiedje, 1979). Despite the vast benefits of CC, further research is needed to better understand the interactions of CC on residual manure-N uptake, mineralization, and immobilization.
Impact of management practices on N2O target gene abundance
Numerous studies have reported changes in the composition of functional microbial communities involved in N2O emissions in response to agricultural practices and environmental factors (Rich & Myrold, 2004; Webster et al., 2005; Dambreville et al., 2006; Philippot et al., 2007; Henry et al., 2008; Hallin et al., 2009). Using RT-qPCR, (i.e., extracting microbial RNA and converting RNA to DNA for gene amplification via qPCR) we were able to quantify microbial target genes responsible for N2O production at the time of sampling. However, we experienced high variability in gene copy number for each of the target genes of interest (e.g., nirK, nirS, and nosZ; Fig. 5). Due to this variability amongst replicates, and despite the high order treatment interaction significance, we did not find sound ecological significance from this microbial analysis relating back to the origin of N2O production. However, there are general conclusions that can be made from these results. First, of the two nitrite reductase enzymes (e.g., nirK and nirS), we found that the nirS gene was 5 times more abundant than the nirK gene irrespective of treatment combination; meaning, that the cytochrome cd1-nitrite reductase enzyme (e.g., nirS) was in greater abundance than the copper nitrite reductase enzyme (nirK), suggesting that these soils may potentially be limited in micronutrients such as copper for catabolic mechanisms. Second, we found that the presence of cover crops in conventional-tilled plots after fall manure application resulted in the lowest gene copies for both nirK and nirS. This finding would demonstrate the potential for soil disturbance via tillage to reduce microbial community sizes. However, we did not find strong evidence for the presence of cover crops to increase the amount of nirK and nirS gene copies, and thus increase N2O fluxes. Furthermore, we did not find nirK or nirS gene abundance to significantly increase after fall manure application. These findings would suggest that there are mechanisms other than denitrification promoting N2O pulses with the presence of cover crops and after manure application. Although we did not find the nirK or nirS gene abundance to greatly increase with the presence of cover crops or fall manure application, as we observed with our N2O flux data (Fig. 1) we did find that the nosZ gene copies were 52 and 279 times less abundant than that of nirK and nirS, respectively (Fig. 5), thereby resulting in N2O being emitted rather than N2O being reduced to N2. This result is supported by related literature where other authors have reported that the quantification of denitrification genes in soils commonly show a lower abundance of bacteria having the nosZ gene compared with those having the other suite of genes coding for the different denitrifying reductases (Henry et al., 2006; Babic et al., 2008; Hallin et al., 2009; Bru et al., 2010). In all, these results suggest that a large fraction of the denitrifier community does not have the genetic capacity to reduce N2O and subsequently always produce N2O during denitrification.
The objective of this study was to determine the impact of tillage regime and CC on seasonal manure-N uptake and gaseous C and N losses (i.e., N2O, CO2, and NH3) in a Zea mays L. silage system in Vermont. Our results indicate the presence of CC have the potential to increase both N2O and CO2 emissions relative to fallow land, regardless of tillage regime and manure application season, yet we did not observe the presence of CC to improve N uptake and subsequent liberation for cash crop utilization. Furthermore, our results demonstrate the inefficiency of no-tillage systems with broadcast manure application to retain inorganic-N on-site by reducing the amount of N entering the soil profile while also promoting greater losses of manure-N via NH3 volatilization. As NH3 volatilization from broadcast application is mitigated when soils are moist or when manure application is followed by rainfall, and as N2O emissions are exacerbated under the same conditions, it may be unlikely to achieve mitigation of these three gasses simultaneously. These findings add to a limited dataset on the impact of multiple agronomic practices on GHG and NH3 emissions and cropping system N uptake. As the no-tillage plots were previously managed with conventional-tillage less than two years before the beginning of this study, further data needs to be collected on how no-tillage with cover cropping practices alter C and N dynamics over a longer temporal scale and whether this effect changes with site history.
Education & Outreach Activities and Participation Summary
Participation Summary:
In August of each year, Borderview Farm holds a 'Crop and Soils Field Day' where farmers, service providers, students, and the general community from the northeast region come to learn about the sustainable farming practices that are being researched on the farm. The entire group is led through the farm with about 15 different talks on farming practices, machinery, alternative cropping systems, soil health, etc. I gave a talk on greenhouse gas mitigation via alternative soil management practices in August 2018.
In February 2019, I collaborated with UVM Extension on a presentation at the Northeast Organic Farming Association winter conference in Burlington, Vermont. Presentation Abstract: "Agricultural management practices can impact nitrogen gas emissions which contribute to a loss of fertility and an addition of greenhouse gases. goCrop, a web based nutrient management tool, is being adapted to include a nitrogen management tool (NMT) that relatively compares the impact of rotation, management practices, weather, and soil type on SOM, PAN, N leaching, and N volatilization. To set the context for a hands-on demonstration of the NMT, results from in-field gaseous nitrogen measurements will be paired with crop and yield quality to provide outcomes of adopting BMPs." Powerpoint presentation is attached below.
My advisor and I are also currently working on publishing this study in the Journal of Environmental Quality.
Project Outcomes
This project has focused on the potential for a combination of multiple agricultural management practices (i.e., no-tillage versus conventional-tillage, cover crops versus no cover crops, and spring or fall manure application to (i) mitigate carbon dioxide, nitrous oxide, and ammonia emissions, and (ii) increase N uptake via cover crop and corn biomass. We expected that a no-tillage system with the presence of cover crops and spring manure application would be the most robust system at achieving these two objectives, although we found that the presence of cover crops was the primary mechanism that increased both carbon dioxide and nitrous oxide emissions. Cover crops likely increased carbon dioxide emissions relative to fallow land due to root respiration, although cover crops offset this emissions by ~30% by capturing atmospheric carbon for biomass production. With respect to cover crops and nitrous oxide emissions, we expect that the presence of cover crops increased this emission due to the input of labile carbon substrates with a low carbon:nitrogen (i.e., easily decomposable). This finding demonstrates the importance of site history and subsequent soil physiochemical properties when considering greenhouse gas emissions from soils. Two years prior to when this field trial was established all plots were managed with conventional-tillage; total carbon and nitrogen content of these soils were 3% and 0.3%, respectively. As these soils were relatively low in both total carbon and nitrogen, it is likely that addition of carbon and nitrogen substrates from cover crop biomass stimulated a microbial priming effect, resulting in greater soil organic matter decomposition and subsequent greenhouse gas emissions. For this reason, further research would be needed to see how soil physiochemical properties change after multiple years of adopting no-tillage and cover cropping practices in order to fully understand whether a no-tillage practice with cover crops mitigate gaseous carbon and nitrogen losses over time. We also found that ammonia emissions are greatest affected by tillage regime, where convetional-tillage reduced emissions relative to no-tillage. Due to the potential for conventional-tillage to increase soil organic carbon losses and thereby reduce soil quality, we recommend alternative manure application methods, such as manure injection, if the objective is to reduce ammonia volatilization without greatly enhancing soil organic carbon losses as carbon dioxide. With respect to crop nitrogen uptake and use-efficiency, we would recommend for farmers to apply manure during the spring rather than the fall as we have demonstrated greater corn nitrogen uptake before corn is sewn (i.e., spring application). In all, adopting a cover cropping, no-tillage, and spring manure application system for corn silage in the Northeast would likely result in greater manure nitrogen utilization along with improving soil health over time.
There is vast information in the literature regarding the benefits of cover crops in agricultural systems. These benefits include increased soil health, reduced nutrient leaching and runoff, reduced erosion rates, and more. However, this work was one of the first studies to identify how cover crops interact with different tillage regimes and nutrient application timing to reduce gaseous nitrogen and carbon emissions. In contrast to our expected results (i.e., the presence of cover crops would reduce both CO2 and N2O emissions), the presence of cover crops increased both emissions. This finding highlighted a major tradeoff when considering the benefits of cover crops in agricultural systems, at least for our field site. From our findings, we did not find strong evidence for biological parameters (e.g., N2O target gene abundance, microbial biomass carbon and nitrogen) to influence changes in emissions under different management practices. For this reason, my advisor and I are now curious about the physiochemical properties that could have influenced our findings. In other words, if we are to continue this study, we would focus further on the chemical and physical properties of the soil profile by analyzing the soil profile to a meter depth for different carbon and nitrogen species and how these species change with depth and time. As the total carbon content of these soils were fairly low, we are also interested to see how these conservational cropping practices would influence soil health after a decade and whether these practices promote carbon sequestration and improved soil tilth over time. Overall, we still promote the literature regarding cover cropping and no-tillage practices to have beneficial properties for cropping systems; however, this study promoted the concept that management practices are not always going to be a one-size-fits-all solution. It is important to have an understanding of the soil conditions (i.e., soil type, soil porosity/texture, organic matter content, etc.) if the goal of cropping management practices is to reduce soil organic carbon losses and begin to restore and maintain soil fertility.