Final report for GNE22-276
Project Information
Agriculture contributes about 18% of all greenhouse gases (GHG) global emissions and 10% of US emissions. Dairy farms are an important source of GHG emissions including enteric fermentation, manure management, and soil management. Methane (CH4) from enteric fermentation is the largest source of emissions with about 45% of the total GHG emissions of the full dairy farm system. Most of the efforts to abate CH4 emissions focus on sources with high concentration of CH4. However, combustion for energy recovery is not feasible in enclosed agricultural systems due to the low concentration of CH4 in those emissions. Engineered biofiltration technology is a potential solution for mitigating emissions, converting CH4 into carbon dioxide (CO2) and water. This work aimed to design a pilot-scale biofiltration system to mitigate CH₄ emissions from dairy livestock facilities' ventilation air. A bank of six biofilters was built to study the effect of process conditions on biofilter performance. CH₄ concentration and CO₂ production were measured at the inlet and outlet of the system to calculate the CH₄ elimination capacity as a performance criterion of the system. Experimental data were gathered under different process conditions for the calibration and validation of a mechanistic mathematical model that was simultaneously developed. Both the experimental analysis and the model validation serves as tools to propose engineered microbial biofiltration technology suitable for implementation in dairy farms, contributing to more sustainable agricultural practices.
The overarching goal of this proposal was to design and test a microbial biofiltration system for the biological oxidation of CH₄-contaminated air from the ventilation systems of dairy livestock facilities. I proposed an experimental approach to investigate the effect of process conditions on the biofiltration system’s performance. In addition, I proposed to use the experimental data as input to a mechanistic process model, which I developed as part of my dissertation, to elucidate the relationships between process conditions, mass transfer phenomena, and the biodegradation kinetics of the system. I pursued the following research objectives:
Objective 1. Demonstrate the feasibility of pilot-scale biofilters to biologically oxidize low-concentration CH₄.
Objective 2. Investigate the effect of different operating conditions on CH₄ biofilter performance.
Objective 3. Identify correlations among operating conditions, the system's mass transfer, and biodegradation kinetics.
Objective 1 included the design and construction of a pilot-scale biofiltration system. The biofilter performance was assessed by quantifying the elimination capacity of CH₄. In Objective 2, I evaluated the effect of CH₄ inlet concentration and inlet air flow rate on the CH₄ oxidation capacity of the system, since those variables were known to influence CH₄ oxidation. To evaluate the effect of process conditions on the mass transfer and biodegradation kinetics of the process in Objective 3, I used the experimental data gathered from Objective 2 as input for a mechanistic mathematical model of the system, which I developed as part of my dissertation. In this way, mass transfer and biodegradation parameters were identified as functions of the process conditions, and correlations were established.
The purpose of this project was to design a pilot-scale biofiltration system for the mitigation of methane emissions from dairy livestock facilities' ventilation air. Dairy farms are sources of GHG such as carbon dioxide (CO₂), methane (CH₄), and nitrous oxide (N₂O), and are responsible for about 50% of CH₄ emissions from anthropogenic sources¹. Enteric fermentation, manure management, and soil management are the greatest contributors of CH₄ emissions in dairy farms². CH₄ from enteric fermentation is the largest source of emissions, accounting for about 45% of the total GHG emissions of the full dairy farm system³. Due to the low concentration of CH₄ in dairy farm ventilation air, combustion for energy recovery or existing methods to separate CH₄ from air⁴ are either unfeasible because of the methane concentration thresholds or require high energy demands to treat such low methane concentrations⁵, which makes them impractical to implement in commercial dairy farms.
Dairy cattle are responsible for 25% and 53% of the total emissions from enteric fermentation and manure management, respectively⁶. Since an optimum ventilation system is needed over time for animal well-being, a low concentration of methane is constantly being released into the atmosphere, generating a significant carbon footprint in the environment⁷. Instead, biofilters can be used to biologically treat the CH₄ emissions from the waste gas, allowing farmers to have a dairy system with less net GHG emissions. Biofiltration has been used mostly for the treatment of odor- or ammonia-contaminated air, but applications for CH₄ mitigation are very limited⁸. CH₄-oxidizing bacteria in CH₄ biofilters use low-concentration methane as a carbon source and oxidize the CH₄ into CO₂ and water, decreasing the Global Warming Potential (GWP) of the emissions by 28–34-fold⁹.
A broad range of CH₄ removal efficiencies (13%–99%) is reported in experimental setups of CH₄ biofiltration¹⁰–¹³, due to the empirical nature of biofilter design, the lack of understanding of the underlying phenomena, and the poor ability to control process conditions. This unpredictable nature of the system limits its applicability in the field⁸. Compaction, clogging, and channeling of the packing media are also drawbacks of the process operation at a larger scale¹⁴. In addition to the operational challenges of the system, the effects of process conditions on mass transfer phenomena and biodegradation kinetics, which are closely related to system performance, need to be studied. Therefore, understanding the relationships between process conditions and their effect on biofilter performance was a necessary step I pursued to design stable and reliable engineered biofiltration systems. Such systems can contribute to a reduction of the net GHG emissions of dairy farm systems towards more sustainable agriculture.
Research
I proposed to perform a series of experiments to evaluate the effect of key process conditions (i.e., CH₄ concentration of the inlet air and inlet flow rate) on the CH₄ biofilter performance. This information also allowed me to identify the effect of the process conditions on the mass transfer and biodegradation kinetics of the system, which I later implemented as correlations in a mechanistic process model of the system that I developed as part of my dissertation.
Objective 1. Demonstrate the feasibility of pilot-scale biofilters to biologically oxidize low-concentration methane.
I built a pilot-scale biofiltration setup consisting of a bank of six pilot-scale biofilter columns connected to a gas system where air and CH₄ were provided. Each of the biofiltration columns was made in-house following an optimized column modular design that we had developed in a previous biofiltration-related project, as follows: the biofilter columns were made of polycarbonate tube with an inner diameter of 0.15 m and a height of 1 m. A three-modular design of the columns was considered. The advantages of having a modular design include the minimization of compaction difficulties¹⁰, an easy packing and unpacking process of the columns¹¹, and more flexibility and quicker response against possible clogging or channeling¹⁴. The standard biofilter packing media for the columns consisted of a 50:50 mix of compost obtained from the Penn State Organic Materials Processing Center (OMPEC) facility and woodchips provided by the Penn State Office of the Physical Plant Grounds Maintenance²¹.
Two different sets, each one with three biofilters, were considered. Set 1 was used for the baseline experiments, and Set 2 was devoted to the experiments related to Objective 2, where different CH₄ inlet concentrations and inlet flow rates were tested. Each set had a pair of mass flow controllers to control the CH₄:air inlet concentration ratio. In addition, each of the biofilters had a rotameter at the inlet to allow for setting different inlet flow rates. Tubing, connections, and adapters were used to connect each of the sets to the CH₄ and air supply, and a humidifier was used to control the moisture content in the inlet gas.
The biofilters require a considerable stabilization period to reach steady-state conditions¹⁴. To account for that stabilization period, I proposed a consecutive building process for the six biofilters. That is, the first batch of the building period was dedicated to constructing and setting up the first set of three biofilters. Once built, the stabilization period began at specified conditions and lasted for about two months. Inlet and outlet air samples were taken twice per week to monitor CH₄ and CO₂ concentrations during the stabilization period and to ensure that the system reached a steady state. While the first set was in the two-month stabilization period, I started the building process and tuning of the second set of three biofilters, which also required a stabilization period of two months to reach steady state. In this way, dead times were avoided, as the two sets of biofilters were in different stages throughout the project timeline, allowing us to test various conditions in each of them.
Objective 2. Investigate the effect of different operating conditions on methane biofilter performance.
Operating conditions such as CH₄ inlet concentration and inlet flow rate have an important effect on the performance of methane biofilters¹⁰,¹¹,²²,²³. Three levels—high, baseline, and low—for each of the variables (CH₄ inlet concentration and flow rate) were tested to determine the biofilter performance. The biofilter performance was determined by measuring CH₄ concentrations in the inlet and outlet air streams of the system. We also monitored the concentrations of CO₂. The concentrations of gases were measured by gas chromatography. CH₄ biofilter performance was analyzed using the elimination capacity. To achieve this objective, I proposed two tasks:
Task 1: Baseline experiment
The first set of three biofilters was built in-house. I operated the first set of biofilters under baseline conditions of CH₄ inlet concentration, corresponding to CH₄ concentrations typically found in average dairy farm ventilation air. A preliminary two-month stabilization period was considered, during which a constant flow of methane-contaminated air flowed through the biofilters. This baseline set operated under the same conditions throughout the entire project timeline. This approach allowed me to evaluate the effect of time and the corresponding possible deterioration of the packing material, such as compaction, clogging, and channeling effects, on the CH₄ oxidation capacity. I took samples from the inlet and outlet air streams of the biofilters 2–3 times per week, and I quantified CH₄ and CO₂ concentrations.
Task 2: Evaluate the effect of CH₄ inlet concentration and inlet flow rate on the methane oxidation capacity
For the second set of biofilters, high and low CH₄ inlet concentrations—relative to the baseline condition—were tested. My hypothesis was that the changes in elimination capacity would be significant between these two levels¹⁰. Four sets of experiments were performed. Aleatory triplicates were considered to average the effects of unknown and uncontrollable nuisance factors in the design. In addition, it is known that process conditions affect and produce changes in the microbial community distribution of the biofilter in the long run¹⁴. Therefore, to avoid cross-influence between consecutive experiments, I ran experiments 5 and 6 under low CH₄ concentration conditions first, since high CH₄ concentrations are more likely to influence subsequent runs. I operated the set of biofilters under low CH₄ inlet concentration conditions until a steady state was reached. During this stabilization period, a constant flow of CH₄-contaminated air flowed through the biofilters, and samples were taken 2–3 times per week to monitor CH₄ concentration changes. Once the system reached steady state, experimental variations in the flow rate were introduced for runs 5 and 6.
Table 1. Experiments under low CH4 inlet concentration
and two levels of air inlet flow rate
|
CH4 inlet concentration |
Low |
Low |
Low |
Air inlet flow rate |
Run 5 |
Low |
High |
High |
Run 6 |
High |
Low |
Low |
For runs 5 and 6, a preliminary two-month stabilization period was considered. Thereafter, each replicates per process condition was held for one month. That is, a run was supposed to last three months in total. Samples were taken 2–3 times weekly from the inlet and outlet air streams, and CH₄ and CO₂ concentrations were quantified.
Once runs 5 and 6 were finished, the CH₄ inlet concentration conditions were switched to the high level, and a two-month stabilization period occurred. Once the system reached a steady state, experimental variations in the flow rate were introduced for runs 7 and 8, and each of the replicates per process condition was held for one month. That is, a run was considered to last three months in total. Samples were taken 2–3 times per week from the inlet and outlet air streams, and CH₄ and CO₂ concentrations were quantified.
Table 2. Experiments under high CH4 inlet concentration
and two levels of air inlet flow rate
|
CH4 inlet concentration |
High |
High |
High |
Air inlet flow rate |
Run 7 |
High |
Low |
High |
Run 8 |
Low |
High |
Low |
Objective 3. Identify the correlations among the operating conditions and the system's mass transfer and biodegradation kinetics.
The experimental design proposed in Objective 2 allowed me to gather significant data, including CH₄ concentration, flow rates, CO₂ concentration, and CH₄ elimination capacity under different process conditions. In addition, as part of my dissertation, I developed a mechanistic model describing the phenomena in the biofilter, considering mass transfer, transport phenomena, and reactions occurring at the biofilm level. To that aim, microorganisms were considered to grow on the surface of a solid medium where the microbial degradation of CH₄ to CO₂ and water follows a Michaelis-Menten-based kinetics²⁴,²⁵. Mass balances for CH₄ and CO₂ were formulated in the gas phase and the gas/liquid interface. The mathematical model consisted of a set of ordinary differential equations solved using Python.
For Objective 3, I proposed to use the obtained experimental data as input for the model to identify the mass transfer parameters and biodegradation kinetics parameters using optimization techniques. Having data under different process conditions allowed me to identify the effect of the process conditions on the values of the process parameters. I hypothesized that the inlet flow rate would have a greater influence on the process parameters, since high flow rates can accelerate compaction problems in the packing material and low flow rates can limit mass transfer in the system. I expected that the outcome of this objective would be the obtention of a correlation for each of the process parameters as a function of the CH₄ inlet concentration and inlet air flow rate.
The following are the outputs and deliverables from the project:
Objective 1. Demonstrate the feasibility of pilot-scale biofilters to biologically oxidize low-concentration CH4.
- Three-modular design column
This design process was a collaborative effort with Shakthi N. Suresh, a recent graduate with a master’s degree in engineering design. The images used in this section were sourced from his thesis, which centered on the specific design of the three modular biofilter columns.
The biofilter column comprised three modules; each module measured 33 cm in length and 15 cm in diameter. These modules were constructed using polycarbonate tubes. The modules were stacked and secured using 3D-printed Polylactic Acid (PLA) flanges at both ends, ensuring an airtight seal with gaskets, screws, and wingnuts. The entry point for the air-CH₄ gas mixture was at the bottom, which then traveled through the packing media enclosed within the modules. The 3D-printed base unit was designed to include an optional drainage feature, while 3D-printed legs offered versatility for standalone placement or mounting on a base to create repeatable units. Figure 1 shows the CAD design for the modular biofilter and the pictures of the prototype in the laboratory.
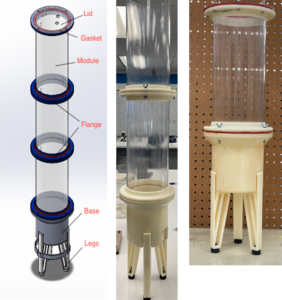
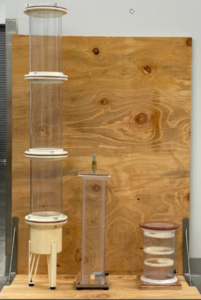
A mixing chamber and a humidifier were also built using polycarbonate tubes and 3D-printed parts. The mixing chamber was designed to appropriately mix the air and CH₄ streams, while the humidifier ensured that the incoming gas mixture was adequately saturated, preventing dryness in the packing material and maintaining optimal moisture levels for microbial growth within the compost. For the final prototype, we mounted it on a wooden structure alongside the humidifier and mixing chamber, presenting an initial design concept for the structure intended to house the nine biofiltration columns (see Figure 2).
Although this initial design was successfully constructed, we encountered several drawbacks during the construction process, particularly in achieving the necessary airtight system critical for our application. This design analysis exposed the limitations of 3D-printed components in maintaining the required airtight integrity for our specific applications. These challenges raised concerns about scaling up to accommodate nine biofilter columns. As a result, we opted to transition to the single-module column design, aiming for a more streamlined and compact configuration while building upon the insights and design lessons gained from our experience with the three-modular column design.
- One-modular design column
This design process was collaborative with Tate Greiger, an undergraduate researcher in the Department of Engineering Science.
The biofilter column body was made from a polycarbonate tube with an internal diameter of 15 cm and a height of 1.2 meters. To cap both ends of the column, we employed Acetal Resin (Delrin®) to machine the lids. To ensure airtight sealing between the polycarbonate tube and the lids, O-ring piston seals were utilized. All additional parts that did not require airtight characteristics were 3D printed. The entry point for the air–CH₄ gas mixture was located at the bottom, and the mixture traveled through the packing media enclosed within the column. A 5 cm chamber was integrated at the base to ensure the uniform mixing of the inlet air–CH₄ stream before it entered the packing material. Within this chamber, a drainage system was implemented to manage potential leachate during biofilter operation. All the fittings used to connect the column with the tubing were push-to-connect fittings suitable for airtight conditions.
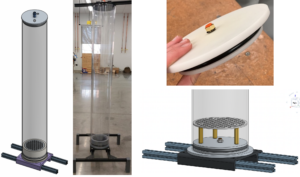
The biofilter column was attached to an aluminum extrusion structure, providing both stability and the convenience of mobility, all within a compact design that was well-suited for easy and efficient scalability. Figure 3 illustrates the various components that made up the biofilter column. To confirm the absence of leaks and validate the suitability of the design for scaling up to nine columns, a leak test was conducted using the bubble test method.
After validating the design's airtight integrity, we built the remaining eight biofilters. To house this bank of biofiltration columns, we designed three aluminum extrusion structures, each capable of accommodating up to three biofiltration columns. This configuration enabled us to efficiently execute the various conditions outlined in our experimental design.
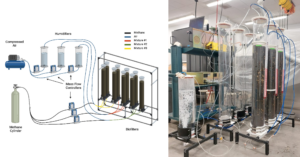
Fittings and tubing were installed in the bank of biofiltration columns, and the system was confirmed to be airtight. Rotameters were used to monitor the inlet flow in each of the columns. Preliminary tests were also conducted to assess the packing material process and evaluate the overall flow conditions once the columns were packed.
This specific objective stands at 100% completion.
Objective 2. Investigate the effect of different operation conditions on the CH4 biofilter performance.
For this objective, The biofilter media consisted of a 50:50 dry weight mixture of compost, obtained from the Penn State Organic Materials Processing Center (OMPEC), and woodchips received from the Penn State Office of the Physical Plant Grounds Maintenance. Compost and woodchips were kept at room temperature under sealed trash cans to maintain original moisture content. Two days before the experiment, the compost was incubated at 37 ◦C and the moisture content was determined with gravimetric methods. On the day of the experiment, the packing material was adjusted to 55% moisture content with nanopore water and then mixed with the woodchips.
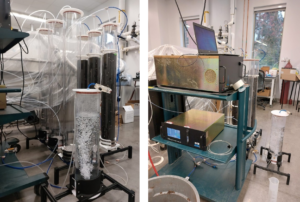
The performance of the biofilters was evaluated by measuring CH₄ and CO₂ concentrations in the outlet gas using Fourier Transform Infrared (FTIR) spectroscopy with a CAI 600 Series analyzer and a multipoint sampling system. The setup allowed automated control of sampling sequences, including purging cycles to prevent cross-contamination. Gas concentrations were quantified in real time using compound-specific absorption peaks. Gas chromatography (GC) was periodically conducted using an SRI 8610C system equipped with FID and ECD detectors to validate FTIR measurements. Outlet gas samples were collected in Tedlar bags and manually injected to confirm the accuracy of FTIR readings.
Figure 6 illustrates the temporal evolution of the CH4 at the outlet of the biofilter (C_CH4,out) during Phase 1, when the system operated at a flow rate of 1 lpm. Subfigure 1.2a shows the performance of biological replicates BF1–BF3 at a target C_CH4,in of around 0.5% v/v, while Subfigures 1.2b and 1.2c present the profiles for BF4–BF6 and BF7–BF9 at approximately CCH4,out of 1.0% and 2.5% v/v, respectively.
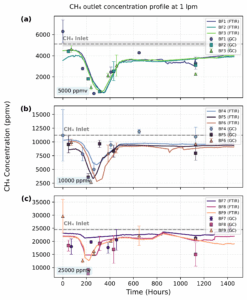
Figure 7
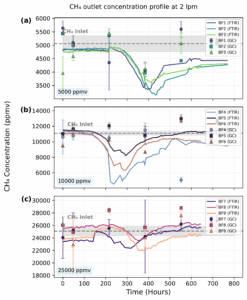
operated at 2 lpm with an air–methane mixture. CCH4,out obtained from FTIR and GC
measurements are shown for each group of biofilters. The dotted gray line indicates the
inlet CH4 concentration (CCH4,in), with shaded areas representing the corresponding standard
deviation.
Figure 7 presents the CCH4,out profiles obtained during Phase 2, in which the flow rate was increased to 2 lpm. As in Phase 1, Subfigures 1.4a, 1.4b, and 1.4c correspond to BF1–BF3 with a target CCH4,in of ∼0.5% v/v, BF4–BF6 ∼1.0% v/v, and BF7–BF9 ∼2.5%v/v, respectively. The evolution of CCO2,out under these conditions is shown in Figure 1.5. Phase 2 experiments were conducted over approximately 800 hours, until no change in the CCH4,in was observed.
The performance of CH4 removal at 1 lpm (Figure 6) and 2 lpm (Figure 7) was evaluated across three CCH4,in using average removal efficiency (RE) and the average elimination capacity (EC) as principal metrics. At 1 lpm, the system exhibited superior RE values compared to 2 lpm, especially under the low concentration condition (5000 ppmv), reaching a maximum near 90%, indicating effective microbial oxidation under low substrate loading. As inflow concentration increased, the RE declined, with 25000 ppmv showing less than 30% removal, likely due to overloading or mass transfer
limitations. These trends align with literature findings, indicating that RE varies significantly, from under 20% to almost 100%, depending on operating parameters and biofilter design.
Conversely, EC increased proportionally with CCH4,in, with 25000 ppmv reaching values close to 20 gm-3 h-1, indicating that a higher CH4 load was processed, despite the decline of efficiency. When the flow rate increased to 2 lpm, RE declined across all conditions, likely due to decreased residence time, whereas EC values increased due to higher methane throughput. Prolonged lag phases before effective treatment were observed at 2 lpm, particularly at 5000 ppmv. A delayed RE peak of approximately 35–40% indicates microbial adaptability or biomass growth before achieving efficient
performance. The data indicate a trade-off between efficiency and capacity; increased flows facilitate enhanced CH4 processing, but this results in reduced removal efficiency (RE), especially under high CCH4,in.
Despite achieving high CH4 oxidation efficiency in the initial stages of the process, the system failed to maintain its performance. After maximum RE was reached, it ultimately stabilized at a suboptimal level, characterized by restricted oxidation capacity and a gradual rise in CH4 concentration.
Unlike the prolonged stabilisation times commonly observed in biofiltration research, the system demonstrated a notably fast methane oxidation capacity. However, this early oxidation capacity was not maintained over time. The system ultimately stabilized at suboptimal removal levels, marked by diminishing efficiency and decreased elimination capacity despite initially promising performance. This decrease may indicate microbial adaptation dynamics, limitations in substrate or nutrients, or inhibitory effects such as biomass overaccumulation.
A two-way ANOVA was performed to assess the impacts of flow rate (1 lpm or 2 lpm), CCH4,in (5000, 10000, 25000 ppmv), and their interaction on the maximum recovery efficiency (RE) and the maximum elimination capacity (EC) under the tested experimental conditions. The assumptions of normality and homoscedasticity were evaluated before the analysis. Both flow rate and CCH4,in had statistically significant effects (p < 0.001) on
RE. Furthermore, a notable interaction effect was seen between flow rate and CCH4,in (p= 0.020), suggesting that the influence of flow on RE was contingent upon the CCH4,in. For the EC, only the concentration had a significant influence (p = 0.036). The influence of rate flow and the interaction between rate flow and initial CH4 concentration were not statistically significant (p > 0.05). For RE, the comparison between 1 lpm and 2 lpm rate flows shows a significant difference, suggesting that flow rate is crucial in recovery efficiency. CCH4,in of 25000 ppmv showed significantly lower RE than 5000 ppmv and 10000 ppmv. Multiple combinations involving 25000 ppmv at 2 lpm exhibited significant differences from conditions with lower concentration or flow rate, confirming a strong interaction effect. Conversely, fewer notable variations were noted with EC. The comparison between
10000 ppmv and 5000 ppmv demonstrate statistical significance, indicating that EC exhibits reduced sensitivity to variations in flow rate or intake concentration.
In summary, over most experimental settings, the overall CH4 elimination efficiency found in this work remained relatively low, despite efforts to maintain stable operational conditions. This outcome is likely attributable to a convergence of characteristics frequently documented in the literature, such as elevated input loads, diminished EBRT, microbial acclimation challenges, and possible mass transfer constraints. Previous investigations indicate that elevated intake concentrations and flow rates may impose kinetic or diffusional limitations, restricting the contact time between CH4 and the active biofilm. Environmental factors, including temperature variations and water accumulation from exothermic oxidation, may have influenced microbial activity and gas transport. The lack of nutrient supplementation may have also limited microbial performance.
Objective 3. Identify the correlations among the operation conditions and the mass transfer and biodegradation kinetics of the system.
I developed a mathematical model. The equations yield a system of partial differential equations. I employed the Finite Element Method in Python to approximate and solve this system of differential equations. Initially, I examined the impact on the airflow by considering low, medium, and high flow levels while keeping the CH4 inlet concentration constant at 9500 ppm (Figure 8). According to the results, lower flow rates result in a more effective removal capacity for the system. This effectiveness, however, is limited at higher flow rates. Furthermore, the production of CO2 follows a similar pattern. This trend also raises concerns about potential compaction issues the system may encounter in the long term, highlighting the importance of avoiding very high air flow rates to prevent such compaction problems.
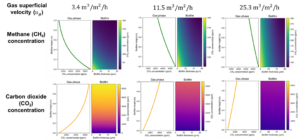
An additional analysis focuses on the influence of packing material type and specific surface area on the system's performance (Figure 8). Figure 8a depicts a standard laboratory-scale biofilter, featuring a blend of 50% organic material and 50% inorganic material, while Figure 8b showcases a biofilter containing compost as the sole organic material, offering nearly 2.5 times greater specific surface area compared to the mixed material scenario. According to the results, an increase in available surface area, facilitating both mass transfer and biodegradation, leads to enhanced methane conversion. Nevertheless, in practical applications, employing solely compost as the packing material is not advisable, as it tends to be prone to compaction and clogging over time. Thus, a balanced ratio of organic to inorganic material in the packing of the filter is a more sustainable approach.
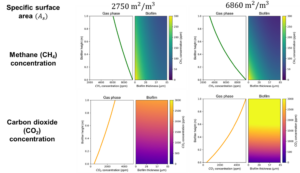
For model calibration and validation, Figure 9 presents the dynamic simulation results of the methane biofiltration system compared with experimental data collected at an inlet flow rate of 1 lpm and an initial methane concentration of 5000 ppmv. The figure illustrates the temporal evolution of key
system variables, including CH4 and CO2 outlet concentrations, CH4 removal efficiency, and biofilm thickness. The simulation results capture the observed trends experimentally, allowing for a detailed analysis of microbial adaptation, substrate limitation, biomass dynamics, and mass transfer phenomena throughout the operational period.
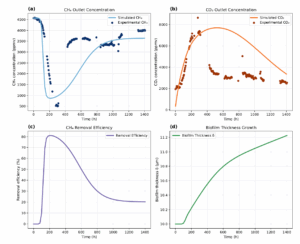
Figure 9(a) shows the simulated and experimental CH4 outlet concentrations over time. Initially, the outlet concentration remains relatively high due to the physiological adaptation lag of the methanotrophic community, despite the high availability of CH4 in the system. As microbial adaptation progresses, methanotrophic activity increases, leading to a rapid consumption of CH4 and a sharp decrease in the outlet concentration. Following this peak, a gradual recovery in CH4 outlet concentration is observed, primarily attributed to substrate diffusion limitations within the biofilm and biomass decay reducing the active microbial population. Notably, the biofilm thickness remained relatively constant throughout the simulation, with an increase of only approximately 1.2 μm (Figure 1.3(d)), indicating that the outlet concentration dynamics were governed by biological factors rather than by significant changes in biofilm growth.
Figure 9(c) shows the CH4 removal efficiency over time. The system exhibits a rapid increase in removal efficiency during the early stages, reaching a maximum of approximately 80% around 200 hours. This initial rise reflects microbial adaptation and high substrate availability. After the peak, removal efficiency gradually declines, with final values around 15% at the end of the simulation period. The simulated maximum removal efficiency exhibited a relative error of approximately 10.2% compared to the experimental value (89.1%). The final efficiency showed a larger relative error of 44.2% compared to the experimental final value (26.88%). Additionally, the model underestimated CH4 outlet concentrations after 400 hours, suggesting a slight overestimation of microbial activity or an underestimation of biomass decay rates during the long-term simulation.
Figure 9(b) shows the simulated and experimental CO2 outlet concentrations over time. The CO2 profile exhibits an initial increase, reaching a maximum around 400 hours, followed by a gradual decline. The early rise in CO2 concentration is primarily driven by methane oxidation, which produces CO2 as a metabolic by-product. As methanotrophic activity peaks, CO2 generation reaches its maximum. Subsequently, the decline in CO2 concentration is associated with reducing active biomass due to substrate limitation and decay processes.
The model also incorporates a basal respiration term associated with living and dead biomass, providing a continuous, low-level contribution to CO2 production even when methane oxidation rates decrease. Notably, the model tends to overestimate CO2 outlet concentrations, particularly after 400 hours, consistent with the underestimation of CH4 outlet concentrations observed in Figure 1.3(a). This behavior suggests a slight overestimation of microbial activity or an underestimation of biomass decay rates during long-term simulation, affecting substrate consumption and by-product generation.
Overall, the model provides valuable insights into the role of microbial physiological adaptation in determining methane removal efficiency. Incorporating an adaptation function allows the simulation to realistically capture the delayed microbial activation observed during startup periods, improving the predictive capability compared to conventional models. Furthermore, the modular structure of the model enables straightforward
extensions, such as incorporating substrate inhibition effects, pH influences, or possible microbial community shifts. These features position the model as a flexible and mechanistic tool for studying and optimizing methane biofiltration systems under dynamic operating conditions.
This project aimed to evaluate and improve the performance of methane biofiltration systems for the mitigation of greenhouse gas (GHG) emissions from dairy ventilation air. Specifically, the goal was to design and test a pilot-scale biofiltration setup and to develop a dynamic mathematical model that would provide a deeper understanding of the key factors affecting CH₄ removal efficiency. By coupling experimental work with mechanistic modeling, I sought to identify operational and biological limitations and create a framework supporting the scale-up and real-world implementation of biofilters in farm environments.
To achieve this, I constructed a modular, pilot-scale biofiltration system consisting of nine biofilter columns operated under a range of flow rates and methane inlet concentrations. I used high-resolution gas analysis with FTIR and gas chromatography to monitor CH₄ and CO₂ dynamics under various operating conditions. The experimental results showed that CH₄ removal performance was strongly influenced by air flow rate, methane concentration, and time-dependent factors such as microbial adaptation and packing media compaction. These findings highlighted common challenges in biofilter operation, including system stabilization, uneven flow distribution, and gradual performance decline.
Building on the experimental data, I developed a dynamic biofilter model incorporating Monod kinetics, mass transfer limitations, and microbial physiological adaptation. The model successfully reproduced the key experimental trends I observed, including the delay in CH₄ oxidation at startup and the trade-off between removal efficiency and elimination capacity under different loadings. More importantly, it provided mechanistic insights into the coupling of gas transport, microbial growth, and biomass turnover—insights that would not have been possible through experiments alone.
By integrating experiments and modeling, I achieved a much deeper understanding of biofilter behavior, which directly supports the scale-up and design of more robust and efficient systems. The model allowed me to identify critical design and operational parameters—such as biofilm thickness, adaptation kinetics, and gas-liquid mass transfer coefficients—that must be considered for scaling up systems to commercial applications.
I fully met the objectives of my project. The combination of experimental results and dynamic modeling enabled me to clarify key mechanisms affecting biofilter performance and to offer practical recommendations for future design and operation improvements.
Although the full-scale implementation on a cooperating farm was beyond the scope of this phase, my findings provide direct support for future deployment. For example, the model predicts that operating at 1 L/min with 5000 ppmv CH₄ could achieve removal efficiencies of over 85%, depending on proper stabilization and moisture management. This demonstrates that the knowledge generated through my project can lead to more sustainable, efficient methane mitigation strategies for agricultural systems.
Education & Outreach Activities and Participation Summary
Participation Summary:
I have shared the results of this proposed work with specialized audiences during scientific conferences and symposia at the local, regional, and national levels, in the form of poster and oral presentations. The shared results include:
- González, C. Methane Biofiltration Systems and Their Engineering Challenges. Microbial Technologies to Mitigate Methane Emissions Cross-track Symposium: Climate Change and Microbes (CCM) ASM MICROBE 2023. Houston-Texas. June 15- 19 (Invited Speaker)
- González, C., Peacock, V., Geiger, T., Vasco-Correa, J. 2023. Air biofiltration technology for the mitigation of diluted methane emissions. Penn State Climate Solutions Symposium. Penn State Institutes of Energy and Environment IEE. May 22-23. Poster.
- González, C., Peacock, V., Geiger, T., Vasco-Correa, J. 2023. Assessment of a bank of biofiltration system for dilute-methane emissions abatement. ASABE Annual International Meeting. Omaha-Nebraska. July 08-12. Poster
The findings of this project are planned to be submitted for peer-reviewed publication. Target journals included Journal of Environmental Management, Environmental Science and Pollution Research, and Journal of Environmental Chemical Engineering.
The proposed research allowed me to explore the further design of the technology and identify potential pathways for future implementation in the field. Outreach to dairy farmers and policymakers was planned for later, once the technology reached a more mature state and was ready for deployment.
Project Outcomes
This project contributed to agricultural sustainability by developing and testing a biofiltration system capable of reducing methane emissions from dairy ventilation air. Environmentally, the system achieved over 85% methane removal under optimized conditions, helping mitigate the impact of CH₄, a greenhouse gas with a global warming potential far greater than CO₂. Economically, the system was built using low-cost, accessible materials such as compost and woodchips and required minimal energy, making it viable for small and medium-sized farms without major infrastructure changes. Socially, this technology offers farmers a practical way to adopt sustainable practices, potentially improving their eligibility for climate-smart incentives, certifications, or carbon markets.
The project also increased understanding of system dynamics through coupled experimental work and mathematical modeling, which together support future scale-up and customization. These results were shared through national conferences and form the basis of an upcoming peer-reviewed publication, ensuring that the knowledge generated can benefit a broader agricultural community and promote long-term methane mitigation strategies in the sector.
Throughout this project, both my advisor and I deepened our understanding of the technical, biological, and operational challenges involved in implementing sustainable agricultural practices, particularly for methane mitigation in dairy systems. Our awareness of the role that microbial processes and engineered systems can play in reducing greenhouse gas emissions significantly increased. This experience strengthened my commitment to advancing sustainable technologies for agriculture, and I plan to continue researching microbial-based solutions for environmental challenges in my future career. The project also generated research outcomes shared through national conferences and is currently being prepared for submission as a peer-reviewed journal article.