Final report for SW16-070
Project Information
The purpose of the research is to determine if soil solarization under Pacific Northwest conditions is a feasible strategy for managing weeds and soilborne pathogens in tree seedling nurseries. We conducted replicated on-farm trials to test the efficacy of soil solarization in commercial tree seedling nurseries in Mima, WA; Boring, OR; and Yamhill, OR. We also conducted a replicated field experiment to examine how soil moisture and solarization duration affect solarization effectiveness. We examined soil solarization effects on viability and germination of four weed species, quantified fall emergence of naturally occurring weeds, measured impacts on populations of soilborne Fusarium and Pythium spp., examined effects on soil microbial communities, and monitored soil temperature, soil moisture, and local weather during the experiments. We demonstrated that soil solarization resulted in crop growth benefits and reduced the time needed for hand weeding by 52-54%. We produced an initial version of an online soil solarization model to help growers forecast the effectiveness and minimum time required to achieve effective soil solarization based on their location and start date. Other outreach activities included four talks to Pacific Northwest grower groups, a field day, a grower workshop, publications in two grower magazines, and thirteen presentations at regional or national scientific conferences.
- Determine if soil solarization is an effective and economically feasible way to control weeds, soilborne plant pathogens and improve tree seedling growth in Pacific Northwest nurseries.
- Optimize soil solarization for Pacific Northwest conditions. Determine optimal solarization duration by evaluating the effect of soil moisture conditions and soil texture on achieved soil temperature.
- Develop a web-based grower-friendly model for predicting the length of time necessary for disinfesting bareroot nursery soil based on geographic location, soil moisture content, and start date.
Cooperators
- - Producer
- (Educator)
- - Producer
- (Researcher)
- - Producer
- (Researcher)
Research
This project tests the hypothesis that pre-plant soil solarization will be a feasible and effective way to manage weeds and soilborne pathogens in Pacific Northwest tree seedling nurseries.
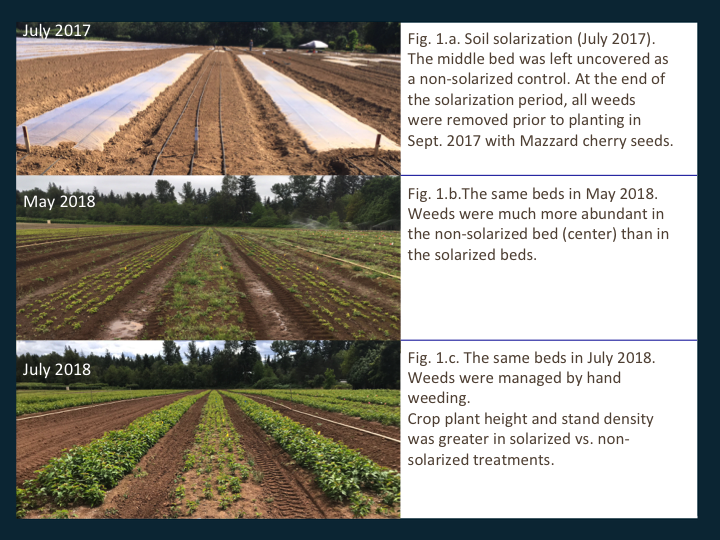
Objective 1: We conducted efficacy trials in three commercial tree seedling nurseries in Mima, WA, and Boring and Yamhill, OR, in 2016 and 2017. In each location, there were two treatments (solarized for 6 weeks or non-solarized) and three replicates per treatment. At Mima, WA we also included a treatment of methyl bromide fumigation in 2016. Individual plots were 30.5 m by 1.2 m. Weed seed packets containing seeds of Poa annua, Polygonum pensylvanicum, Amaranthus retroflexus, and Portulaca oleracea were buried in each plot at 5 and 10 cm depths. Composite soil samples containing indigenous soilborne pathogens and other microbes were placed in sachets and buried at 5 and 15 cm depths. After the trial, weed seed germination and viability were tested in the lab. Weed emergence was counted in 0.25 m2 plots the following fall and spring. We also determined the time required for field laborers to hand weed solarized and solarized plots at the two Oregon locations in 2018. Plate counts of Fusarium spp. and Pythium spp. were determined from the composite soil after the trial, and DNA was extracted for subsequent analysis of fungal, oomycete and bacterial communities with Illumina MiSeq. Soil temperature and moisture were monitored continuously with reflectometers attached to a datalogger. Weather stations at each site measured air temperature, wind speed, and solar radiation. Crop growth was monitored in the year after solarization (2017 and 2018). Crop parameters included stand density, seedling height and stem caliper. Soil samples were collected from the top 15 cm of soil in all plots before and after solarization and brought to the Central Analytical Laboratory at Oregon State University for nutrient analysis. Soil pH was measured using a pH probe, total carbon and nitrogen were assessed using a dry combustion method (Elementar), and nitrate and ammonium were extracted from soil with a 2 M potassium chloride extractant and measured with a Lachat flow injection analysis system.
Objective 2: Field studies. In 2016 at the Boring site, we conducted a factorial experiment designed to determine optimal soil moisture conditions and minimum duration for effective soil solarization (Fig. 1). Four soil moisture levels (low = 13%, medium = 17%, high = 23%, and very high = 25% volumetric water content) roughly corresponding to initial values of 56, 73, 100 and 108% field capacity at the 5 cm depth were achieved with drip irrigation under the plastic, and compared over 3 different durations of solarization (3, 6, and 9 weeks) or a nonsolarized control. Pathogen populations, weed seed viability, weed emergence and crop growth were quantified as described in Objective 1. Pythium spp. and Fusarium spp. were quantified from the composite soil samples buried at 15 cm through dilution plating. DNA was extracted from soil buried at 5 cm for subsequent analysis of soil oomycete, fungal, and bacterial communities. To determine if soil solarization has a lasting impact on soil microbial communities, we collected soil in April 2017, seven months after the solarization trial, soon after tree seedlings in these plots emerged. We repeated the soil moisture by solarization duration factorial experiment in 2017 but unlike 2016, treatments were completely randomized to reduce error associated with non-uniform distribution of soilborne pathogens and weeds. Similar soil moisture levels were achieved as for the previous year: low = 15.4%, medium = 18.9%. high = 20.4%, and very high = 25.5% volumetric water content (5 cm depth).
Controlled environment studies.To better understand the effectiveness of soil solarization in the PNW, a controlled study using local weed species and soilborne pathogens was conducted to determine viability of weed seeds or pathogen inoculum following exposure to high temperatures for different time periods. The objective was to determine the exposure time and temperature required to cause mortality to aid in developing models that predict species-specific thermal susceptibility. Weed species included annual bluegrass (Poa annua), common purslane (Portulaca olearacea), Pennsylvania smartweed (Polygonum pensylvanicum), and redroot pigweed (Amaranthus retroflexus). Pathogens included Fusarium oxysporum, Pythium ultimum, Phomopsis sp., Ilyonectria sp. and Agrobacterium tumefaciens.
Twenty-five seeds of each weed species were imbibed on saturated germination paper for 4 h before placing in the heat treatments. Three temperature treatments, 45, 50, 55 ºC, were chosen from the range of reported temperatures in the top 5 cm of the soil profile during the field solarization trials. The capsules enclosing the imbibed seeds were incubated at a constant temperature. The incubation continued until a species reached 100% seed mortality for each temperature or for 336 h. The trial was repeated. After treatment, viability of ungerminated seeds was assessed using the tetrazolium staining method.
Pathogen isolates were obtained from non-solarized plots at the Clackamas County site and identified using Sanger sequencing of DNA. Inoculum of fungi and oomycetes was produced on sterilized wheat seeds inoculated with mycelial plugs and allowed to grow at room temperature for 14 days. Ten grains of wheat seed inoculum were placed on water-saturated germination paper inside lidded plastic capsules and plated in constant temperature incubators at 45, 50, and 55 ºC. The crown gall pathogen, Agrobacterium tumefaciens (rinsed cells), was added to moistened, sterile sand in lidded capsules. Three replicate capsules were removed from each temperature treatment per exposure period, and refrigerated at 4 ºC for up to 24 hours until plating onto selective media. Pythium ultimum inoculum was plated onto PARP, and fungal inoculum was plated onto Komada’s medium. Agrobacterium was dilution plated onto YEP medium. Inoculum survival was assessed by scoring each grain for hyphal outgrowth (fungal or oomycete) or counting colonies on selective media (bacteria).
Objective 3: Development of a mathematical model that is applicable across soil textures and solar irradiation conditions (climate and latitude) necessitates that we first quantify the heat transfer coefficient across the air-plastic-soil interface. Detailed monitoring of heat transfer will be performed using a subset of plots at the research site. Data will be used to validate and if necessary fine-tune the “to-be-modified” HYDRUS model to simulate the heat transfer in the soil under plastic mulch (Fig. 2).
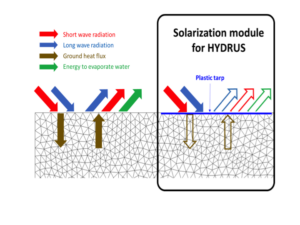
In 2017, two 10 m by 6 m plots with three insets of 80 cm by 30 cm deep holes (instrumented locations) were prepared (Fig. 3). One plot was covered with a solarizing plastic sheet and the other plot was left as bare soil. Each inset was packed with soil sieved through 2 mm. This level of homogeneity is required to guarantee good soil contact with instruments installed for monitoring soil temperature, soil heat flux, and soil moisture.
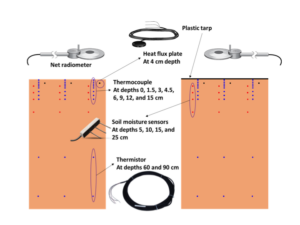
A total of 48 thermocouples monitored soil temperature at depths 0, 1.5, 3, 4.5, 6, 9, 12, 15 cm for three replicates in each of the two plots. Decagon GS3 sensors were used to monitor soil moisture and temperature at depths 5, 10, 15, and 25 cm for three replicates in each of the two plots (24 total). Two thermistors were installed at 60 and 90 cm depths in each plot to monitor the deep soil profile temperature. Heat plates were installed at the 4 cm depth for all three replicates in each plot to measure soil heat flux and soil thermal diffusivity. A net radiometer was installed in each plot to monitor the net radiation above both the bare soil and the plastic sheet. Ambient air temperature was measured in the bare soil plot.
Both plots were monitored for few days prior to solarization and the data will be used to compare the soil data and net radiation between the two plots. The solarization plot was then covered with plastic for a period of two weeks to compare the soil temperature and heat flux between solarized and non-solarized plots under dry soil conditions.
It is known that soil thermal properties are changed by moisture. Therefore, the plastic cover was removed after 2 weeks and the soil moisture of both plots brought to field capacity using a sprinkler irrigation system. Upon completion of irrigation, the solarization plot was recovered with plastic to compare the soil temperature and heat flux between the solarized and non-solarized plot under wet soil conditions.
Objective 1: A summary of 2016 and 2017 soil temperature data is shown in Fig. 4. For all sites and both years, solarization successfully increased the maximum soil temperature at 5 and 15 cm depths, as well as the thermal hours over 35°C. The increase at Mima was less than at the other two sites. Cloud cover strongly suppresses soil temperature; 2016 had more cloud cover and was colder than the average, whereas, 2017 was warmer than the average. Comparing both years provides an idea for the range of effectiveness of this method on soil temperatures, clearly demonstrating that even during cold years, solarization significantly increases soil temperatures.
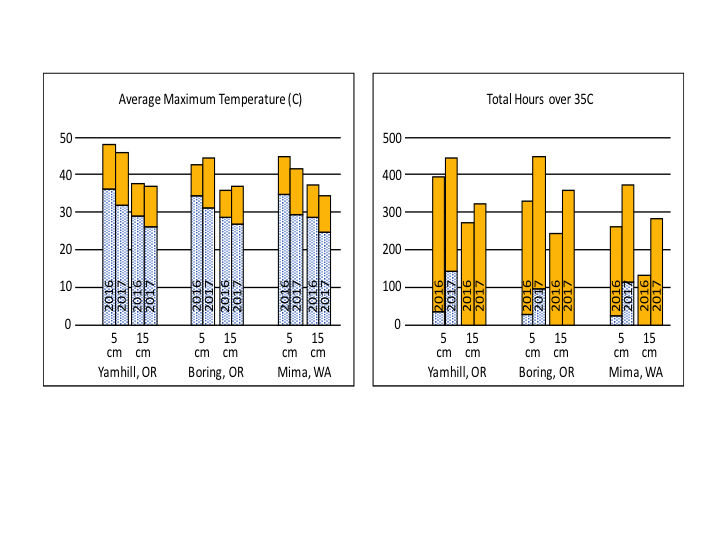
For both years, at all sites and depths, solarization was most effective on Polygonum pensylvanicum, least effective on Portulaca oleracea, and resulted in increased dormancy in Amaranthus retroflexus. In 2016, more Poa annua seeds survived at 10 cm in solarized plots than in non-solarized plots, while in 2017, solarization successfully reduced seed viability at all depths (Fig. 5).
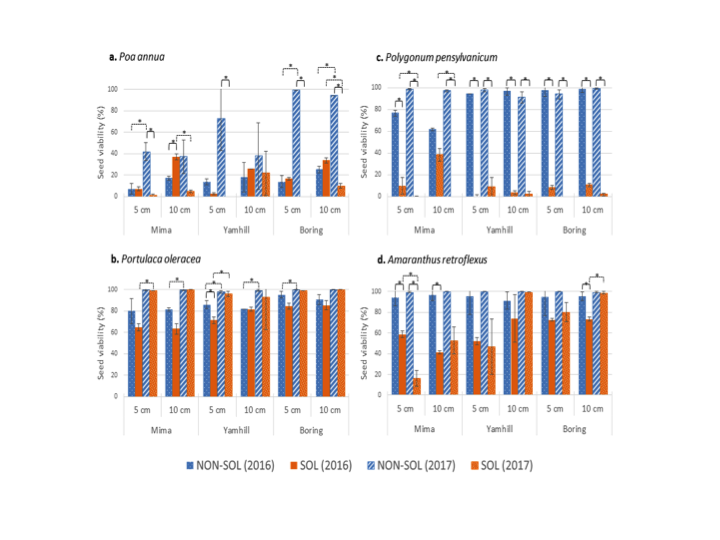
The density of emerging fall and spring weeds was reduced in solarized beds as compared to nonsolarized beds. For example, at Boring in 2016, fall weed seedling density was 0.21/0.25 m2 in solarized beds as compared to 5.26/0.25 m2 in non-solarized beds. The season-long time required for labor crews to hand weed plots was reduced by 52-54% in solarized beds compared to nonsolarized beds at Boring (Clackamas Co.) and Yamhill (Yamhill Co.), respectively.
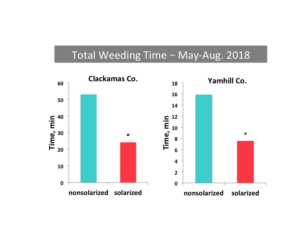
For both years, at all sites, Fusarium spp. and Pythium spp. were not recovered from solarized plots from the 5 cm (=2") depth. Both pathogens were recovered from the 15 cm (=6") depths but populations were generally reduced by solarization. Results for 2017 are shown in Fig. 7.
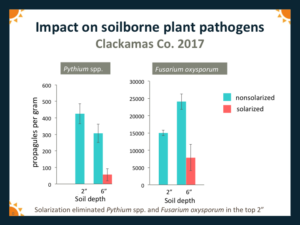
Soil solarization had significant effects on the soil microbial community, especially at the 5 cm depth. Diversity of bacteria, fungi and oomycetes was reduced by solarization, although populations of certain microbes increased following solarization. Changes to the relative abundance of bacteria at one site is shown in Fig. 8.
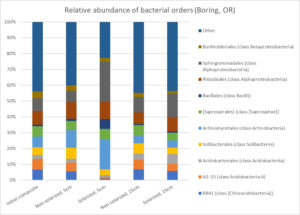
Crop growth in the year following solarization was generally significantly greater than in nonsolarized plots. For example, at the Boring site in 2018, average height of Mazzard cherry seedlings grown in solarized plots was 76% greater than for seedlings in non-solarized plots (Fig. 8). At Yamhill in 2018, average height of apple seedlings in solarized plots was 54% greater, and stand density was 24% greater, than for seedlings grown in nonsolarized plots (Fig. 9).
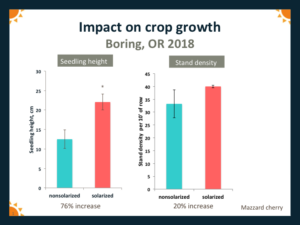
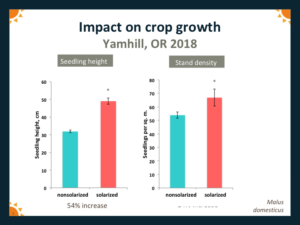
Objective 2: Field study. The moisture by duration experiments showed strong difference between the 2016 and 2017 year with respect to the effect of moisture content on the total hours achieved over 35°C. In 2016, the highest impact was seen for medium moisture contents, but this is not as clearly seen in the 2017 data (Fig. 10).
There was a strong effect of soil moisture on the duration of solarization required to suppress weeds. In 2016, a 3-week solarization period was just as effective as 6- or 9-weeks of solarization as long as the soil moisture level was high or very high (Fig. 11). This suggests that solarization effectiveness is not simply a function of soil thermal hours, which was highest in the medium soil moisture treatment. These results also indicate the potential feasibility of solarizing soil in Pacific Northwest cropping systems when the summer fallow period between crops is short.
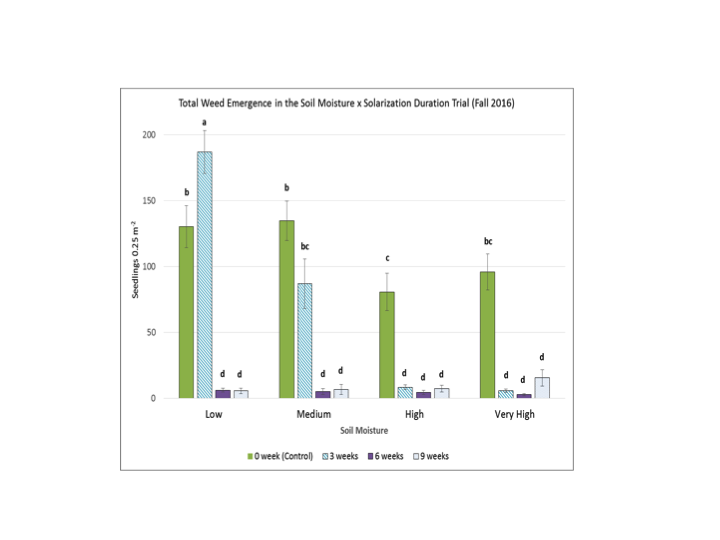
Soil moisture did not have a strong effect on Pythium spp. or Fusarium spp. but all solarized soil had significantly lower populations of each group of organism than initial composites, even after 3 weeks of solarization.
The Illumina Mi-Seq analysis of the bacterial communities indicate differences between communities in the three solarized treatments vs. the non-solarized treatment, and between low moisture vs. all other moisture levels. Treatment differences in bacterial communities were evident seven months after the solarization trial ended.
Controlled environment study. In the controlled environment study, mortality data were fitted to the 2-parameter Weibull model. For each temperature treatment, the time required to kill 90% of seeds tested was determined using the summary and estimated effective dose functions. The thermal death curve of each species was constructed based on the equation. The LD50 differed among species for each temperature treatment. Among the four species, annual bluegrass was most sensitive with an under 10 h at 50 ºC. Pennsylvania smartweed and redroot pigweed required 10 h at 55 ºC to have a similar response. Common purslane was the only species that did not reach complete mortality in the 45 and 50 ºC treatments.
All pathogen isolates included in these trials exhibited temperature sensitivity, and some isolates were more tolerant of high heat than others. Estimates indicate that Fusarium oxysporum was killed with 65.2 hours, 14.4 hours, or 4.6 hours of constant exposure to temperatures of 45, 50, or 50°C, respectively. In contrast, Pythium ultimum isolate was killed with 81.1 min, 35.6 min, or 16. 5 min at the same temperatures, respectively. Agrobacterium was killed after exposure for 1 hour at 50°C or 16 hours at 50°C. Mortality curves were used in the development of the online solarization model to predict the effectiveness of soil solarization based on exposure to soil temperatures.
Objective 3:
We released an initial version of the online soil solarization model and modified it to include additional weed species and pathogens https://uspest.org/soil/solarizeV2beta1 Growers can select the weather station nearest to their farm and choose intended start and end dates for solarizing. The model output includes the predicted temperatures at 3 soil depths, and forecasts the length of time required to solarize to kill soilborne Phytophthora species (P. ramorum and P. pini), three weed species, and one pathogen species. The model was quite good at predicting actual soil temperatures in the 3 field trials conducted in 2016 but less accurate when predicting the 2017 data set. An intensive field-laboratory was instrumented to obtain detailed thermal data. The data shows a phenomenon of deep soil heat storage associated with periodic storm patterns that results in two distinct air-soil temperature calibration curves (Fig. 13). Soil thermal dynamics are being analyzed to determine if this hysteretic calibration curve (which depends on antecedent soil heating) will improve the predictive model capabilities since accounting for the amplitude of the hysteretic loop could improve prediction error by at least 5 C.
The second advance toward model improvement is the identification of a parameter set to determine the earliest possible date for solarization. The two parameters are the seasonal soil temperature at 10 cm and the solar irradiation. Soil temperature changes diurnally and seasonally. Regardless of the level of solar irradiation, it was found that the seasonal soil temperature at 10 cm depth had to reach a minimum of 22 C before solarization could be used. This value determines the earliest spring date and latest fall date for applying solarization. This constraint only requires regional knowledge of soil temperature at 10 cm depth which is commonly available from the network of weather stations. The parameter associated with minimum solar irradiation is still being developed.
Interactive sessions were conducted with selected growers to identify common problems with the online tool, leading to improvements to the user interface and model output. Future work will improve the model of soil heating for more accurate forecasting, include grower workshops to train users in how to use the online tool and include additional targets of soil solarization such as weed species and other soilborne pathogens.
Research activities in 2019 were dedicated to data analysis, theoretical developments, and theoretical and numerical model development. The goal is to develop modeling tools that will help growers apply solarization effectively and with minimal impact to other agronomic activities. To that end, efforts were to develop tools to determine the earliest application date and shortest duration. This year’s activities focused on data analysis to elucidate soil’s response to thermal inputs, including an improved understanding of soil thermal function and the development of useful models in support of grower efforts. Results described below.
Web Model: This predicts soil temperatures based on solar irradiation, soil texture and soil moisture. It uses a pathogen response to predict pathogen mortality to a given soil temperature. The model is intended to be both a predictive tool and tracking tool for growers. The model requires a good understanding of a pathogen’s response to soil temperatures. Model for pathogen response is described in #2 below.
Field Mortality Model: The ambition of this model is to predict the response of pathogens and weed seeds to field temperatures. Current strategy uses a technique of counting total daylight hours above a specific temperature to calculated pathogen mortality. But, pathogens and weed seeds have a non-linear response to temperature exposure. Furthermore, present models used to predict seed responses are based on laboratory data where pathogen/seed are exposed to constant temperatures, whereas field exposure is variable. A new modeling approach was developed (logic response linearization) and from that a new model developed, the Field Mortality Model, that can predict pathogen/seed response to field temperatures based on diurnal temperature variability and the accumulation of destructive impact across multiple days. This new model includes the potential for lower temperatures predisposing seeds to damage once higher temperatures are reached (ta), and the potential for recovery of pathogen populations during lower temperature periods (x). This model advances scientific understanding in the field of solarization. Model results are supported by field data. (Manuscript submission for March 2020).
The symbol “i” represents a time increment, and runs from 1 to n, where n = 24hr/Dt, where Dt is the data time interval (e.g., 30 min). Integral limits, Tcrit is the temperature below which no damage is expected. The temperature Tmax is the maximum temperature reached in the day. Before mortality can accrue, a minimum activation time must be exceeded (ta), which is temperature dependent. Figures 1 and 2 depict the process of activating Eq. 1.
SOL-Track: Model for Tracking Solarization Progress in-situ: The model estimates daily mortality using only one data point, that day’s maximum soil temperatures at 5 cm depth. This model was developed from understanding gained by using the Field Mortality Model (described in #3 above). Using the field data obtained from that project, it was noted that solarization will only be effective on days when the maximum soil temperature reaches or exceeded a specific value, here called the solarization threshold temperature. An example of this mechanism is shown in Figure 1, for a weed seed (Poa annua). An important temperature threshold is seen between 50C and 51C: A single degree of temperature increase corresponds to shortening the necessary solarization period from 44 days to 8.5 days (calculating to a target survival value of 1%). This dramatizes the disproportionate effect of high temperatures, and the value of tracking the in-situ temperatures achieved, especially if shortening the solarization period is of value. A second point is the comparison between laboratory expectations and field reality. Maximum daily temperatures below 49°C, although successful in the laboratory, will not result in solarization success in the field (for this seed) because the minimum activation time (ta) is longer than the length of daytime. A third point is the large increase in exposure needed in the field relative to laboratory times. For example, for 50°C, in the laboratory mortality was achieved in about 8 days (Fig. 4), whereas in the field, because of the discontinuous and sinusoidal exposure, 44 days each with a Tmax value of 50°C would be needed to incur the same mortality rate.
This model demonstrates the possibility of customizing solarization durations towards the shortest possible duration, simply by measuring the daily Tmax at 5 cm depth. The value of the SOL-Tracker model is that it opens the door for using solarization for very short periods of time, which is a critical advantage in the Pacific Northwest. (Manuscript in preparation, submission planned June 2020.) Applying the SOL-Tracker model to our field soil temperature data yield results supported by weed survival field data results. However, more tests are needed before it is ready for dissemination. Discussions are underway with a new collaborator to test the model more rigorously at the North Willamette Research Station.
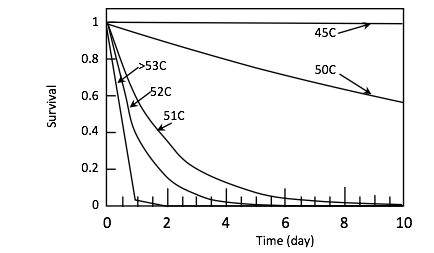
Hysteretic response to solar radiation: Field data analysis revealed the important role of thermal storage at intermediate soil depths (between the diurnal and seasonal zero-flux depths). The short time-constant for this heat storage (order of a week), causes the soil temperature data to exhibit a hysteretic relationship with air temperature (i.e., local weather). Three continuous days of hot or cold weather will change the overall heat flux direction within the soil at the depth of about 50 cm. For example, a one-day storm following a long hot spell, will reduce soil temperatures only for that one day. The reduction is linear. However, if the storm lasts longer than 3 days, it results in a suddent drop in the offset (y-intercept) of the linear response by ~5°C (Fig. 5 orange region to blue or green region). Likewise, it takes 3 subsequent hot days to bring the linear response back up.
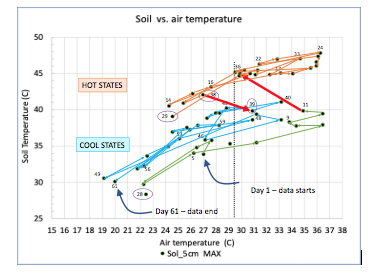
Pursuing this mechanism of deep thermal storage controlling solarization temperatures is a necessary next step to further improve the science of solarization and relevant predictive models. This project has provided the foundational data to explore this mechanism. (Manuscript reporting on hysteretic behavior is in preparation, planned submission August 2020).
Soil solarization in the Pacific Northwest can be very effective at reducing weed emergence and improving the growth of fall-sown tree seedlings. Reduced emergence of winter and summer annual weeds during the growing season following solarization resulted in approximately 50% less time required for hand-weeding, resulting in large potential labor savings for growers. Solarization is most effective on annual weeds, although not all species (such as common purslane) are sensitive to solarization. Soil solarization appears to be effective for reducing weed emergence over a wide range of soil moisture conditions although excessively dry or wet soil conditions during solarization should be avoided. Initial soil solarization trials were conducted beginning in early July for nine weeks, but subsequent trials showed that solarization for as little as three or six weeks was adequate if timed to coincide with clear, sunny weather in summer. Improvements to crop growth following soil solarization generally included greater seedling stand density, likely resulting from suppression of soilborne damping-off pathogens and alterations of soil microbial communities. An online model at https://uspest.org/soil/solarizeV2beta1 is designed to help growers in WA, OR, and CA forecast the length of time needed to solarize soil for management of several weed species and soilborne pathogens based on their location and start date. Soil solarization offers opportunities for nursery growers as well as organic growers of other crops to manage weeds with reduced inputs of chemical pesticides and to save on labor costs.
Research Outcomes
Education and Outreach
Participation Summary:
Online tool for growers and agricultural professionals (1)
Coop, L. B., D. Upper, F. Funahashi, and J. Parke. 2016. Online Soil Solarization Program – for using transparent anti-condensation plastic film to manage two soil-borne plant pathogens: Phytophthora ramorum and P. pini, developed for nursery beds. Version 0.91. Oregon State University Integrated Plant Protection Center Web Site:
<http://uspest.org/soil/solarize > [first version online June 2016]
<https://uspest.org/soil/solarizeV2beta1> [second verson online June 2019]
Trade journal articles (2)
Mallory-Smith, C., Wada, N., and Parke, J. 2019. Here comes the sun. Digger 63:33-36. http://www.diggermagazine.com/here-comes-the-sun/
Parke, J. L., Mallory-Smith, C., Dragila, M., Hill, B., Wada, N., Weidman, C., Coop, L., Buckland, K. 2018. Soil solarization – a potential tool for organic growers to manage weeds and improve soil health. Organic Farmer 1(4):12-18. https://www.yumpu.com/en/document/fullscreen/62280511/organic-farmer-dec-jan-2019
Field days (2)
On-farm field day. Soil solarization as an integrated pest management tool in tree seedling nurseries in the Pacific Northwest. June 28, 2017. Boring, OR. 40 attendees.
Talk and demonstration. Soil solarization for native plant nursery growers. Sept. 9, 2018. San Rafael, CA. 45 participants.
Grower workshop (1)
Half-day grower workshop and demonstration on soil solarization at the N. Willamette Research and Extension Center, Aurora, OR. October 29, 2018. 42 participants.
Video (1)
Soil solarization in the Pacific Northwest. 2017. Video (3:06 min). https://youtu.be/-Ke_ucsBzgw
Online newsletters (2)
Soil solarization in the Pacific Northwest. 2017. Feature Story. Western IPM Center. September issue. http://westernipm.org/index.cfm/ipm-in-the-west/agriculture/soil-solarization-in-the-pacific-northwest/
Soil solarization in the Pacific Northwest. 2017. Feature Story. Western SARE Winter Newsletter. [tba]
Talks for growers and agricultural professionals (9)
Doane, S. 2016. What’s Going Down? How to reduce off-target losses and lower pesticide risk using decision support tools. Oktoberpest: Pest Management Workshops for Greenhouse and Nursery Growers. Going Soft: Designing Target-Focused IPM Programs. Oct. 6, 2016. N. Willamette Research and Extension Center, Aurora, OR. Oral presentation. 25 attendees.
Doane, S. 2017. Soil solarization trials at J. Frank Schmidt and Son, Co. Nursery. Clackamas River Basin Council. Mar. 16, 2017. Oral presentation. 30 attendees.
Elliott, M.E., and Parke, J. L. Preventing Phytophthora contamination in native plant nurseries. May 9, 2017. Research and Extension Center, Washington State University-Puyallup, Puyallup, WA. 19 participants.
Parke, J. 2016. Soil solarization for management of pathogens and weeds. Integrated Pest Management for Nursery, Reforestation, and Restoration Programs. Joint annual meeting of the Western Forestry and Conservation Nursery Association and the Intermountain Container Seedling Growers’ Association, Sept. 14-15, 2016. Troutdale, OR. Oral presentation.110 attendees.
Parke, J. L. 2017. The state of Phytophthora management: a view from the nursery. Phytophthora Species in Restoration Nurseries, Plantings, and Wildlands II. San Jose, CA. May 18, 2017. Invited presentation. 145 participants.
Parke, J. L. 2018. Plot size influences effectiveness of solarization to control soil Phytophthoras. Invited talk. Phytophthoras in Native Habitats Work Group. Albany, CA. June 21, 2018. 45 participants.
Parke, J. L. Soil solarization in field nursery production systems. Shade Tree Growers Meeting, N. Willamette Research and Extension Center, Aurora, OR. Mar. 7, 2019. 23 participants.
Parke, J. L. 2019. Putting the sun to work: soil solarization for management of weeds and soilborne pathogens. New Mexico Sustainable Agriculture Conference. UNM Valencia Campus, Los Lunas, NM. Dec. 10, 2019. 64 participants.
Parke, J. L. and Elliott, M.E. 2017. Preventing Phytophthora contamination in native plant nurseries. Apr. 26, 2017. N. Willamette Research and Extension Center, Aurora, OR. 13 participants.
Presentations at scientific meetings (15)
Bennett, L., Peterson, E., and Parke, J. L. 2019. Determining the minimum treatment area and importance of soil moisture for effective soil solarization in nurseries. Seventh Sudden Oak Death Science Symposium, June 25-27, 2019, San Francisco, California.Oral presentation.
Dragila, M. 2019. W3188- Soil Physics Working Group, Soil, Water, and Environmental Physics Across Scales, Presentation and Report, January 6, 2019 University of California at Riverside, CA.
Dragila, M. 2020. W3188 – Soil Physics Working Group, Soil, Water, and Environmental Physics Across Scales, Presentation and Report, January 2, 2020, Desert Research Institute, Las Vegas, NV,
Funahashi, F. and Parke, J. L. 2016. Development of a predictive model to estimate the effect of soil solarization on survival of soilborne inoculum of Phytophthora ramorum and Phytophthora pini. Sixth Sudden Oak Death Science Symposium, June 20-23, San Francisco, CA. Poster presentation. 85 attendees.
Mallory-Smith, C., Wada, N., Parke, J. 2018. Weed control in Pacific Northwest tree nurseries using soil solarization. Oral presentation. 18th European Weed Science Society Symposium. June 17-21, Lubljana, Slovenia.
Mallory-Smith, C., Parke, J., Coop, L., Nackley, L., Dragila, M., Wada, N, Hill, B., Weidman, C. 2019. Enhanced implementation of the online soil solarization forecasting model. Western Society of Weed Science, Denver, CO. March 13, 2019. 50 people.
Parke, J., Coop, L., Dragila, M., Funahashi, F., Hill, B., Kandelous, M., Mallory-Smith, C., Nackley, L., Wada, N., and Weidman, C. 2018. Soil solarization for integrated pest management in the Pacific Northwest (USA). Poster presentation. 9th International IPM Symposium. Mar. 19-22, Baltimore, MD.
Parke, J. L., Funahashi, F., Weidman, C., and Peterson, E. K. 2016. Relative heat sensitivities of certain Phytophthora spp. and the potential for soil solarization to disinfest nursery beds in west coast states. Sixth Sudden Oak Death Science Symposium, June 20-23, San Francisco, CA. Oral presentation. 85 attendees.
Parke, J., Mallory-Smith, C., Dragila, M., Hill, B., Wada, N., Weidman, C., Nackley, L., Coop, L., and Funahashi, F. 2018. Soil solarization for managing weeds and soilborne plant pathogens in tree seedling nurseries in the Pacific Northwest. Poster presentation. SARE Our Farms, Our Future Conference. April 3-5, St. Louis, MO.
Parke, J. L., Mallory-Smith, C., Dragila, M., Hill, B., Wada, N., Weidman, C., Nackley, L., Coop, L., Funahashi, F. 2018. Soil solarization for managing weeds and soilborne pathogens in tree seedling nurseries in the Pacific Northwest. Poster presentation. ASHS Annual Meeting, July 30-Aug. 2, 2018. Wash. D.C.
Redekar, N., Trammell, C., and Parke, J. L. 2018. Solarization effects on the soil microbiome at an organic vegetable farm in the Pacific Northwest (USA). Poster presentation. Intl. Congress of Plant Pathology, July 29-Aug. 3, 2018, Boston, MA.
Wada, N., Parke, J., and Mallory-Smith, C. 2017. Soil solarization for weed control in Pacific Northwest field nurseries. Western Society of Weed Science Annual Meeting, March 13-16, 2017. Coeur d’Alene, ID. Oral presentation. 25 attendees.
Wada, N., Parke, J., Berry P.A., Bobadilla, L.K. and Mallory-Smith, C. 2018. Comparison of Solarization and Biosolarization for Weed Control in a Tree Seedling Nursery in Western Oregon. Western Society of Weed Science Annual Meeting, March 12-15, 2018. Garden Grove, CA. Poster presentation.
Wada, N., Parke, J., Berry P.A., Bobadilla, L.K. and Mallory-Smith, C. 2018. Soil Solarization in Oregon: The Impact of Solarization Duration and Soil Moisture on Weed Control in a Tree Seedling Nursery. Western Society of Weed Science Annual Meeting, March 12-15, 2018. Garden Grove, CA. Oral presentation.
Weidman, C.S., Redekar, N. R., Eberhart, J. L., and Parke, J. L. 2018. Impacts of solarization on the soil microbiome in Pacific Northwest tree seedling nurseries. Poster presentation. American Phytopathological Society, Pacific Div., June 25-27, Portland, OR.
Weidman, C. S., Moore, A. D., and Parke, J. L. 2018. Soil nutrient responses to soil solarization. Poster presentation. Oregon Society of Soil Scientists, Winter meeting, Feb. 28-Mar. 2, Corvallis, OR.
Publications (1)
Funahashi, F. and Parke, J. L. 2018. Thermal inactivation of two Phytophthora species by intermittent versus constant heat. Phytopathology 108:829-836.
Theses (3)
Hill, B. C. 2019. Solarization in the Pacific Northwest: A Mathematical Field Model for Accumulation of Linearized Mortality from Variable Intermittent Heat. M.S. thesis, Oregon State University. 112 pp.
Weidman, C. S. 2019. Soil Solarization in Pacific Northwest Tree Seedling Nurseries: Effects on Soilborne Plant Pathogens, Soil Nutrients and the Soil Microbiome. M.S. thesis, Oregon State University. 252 pp.
Wada, N. 2019. Soil Solarization for Weed Management in Tree Seedling Nurseries in the Pacific Northwest. M.S. Thesis, Oregon State University.